An overview of remote monitoring methods in biodiversity conservation
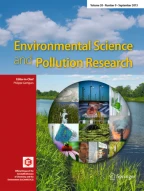
Conservation of biodiversity is critical for the coexistence of humans and the sustenance of other living organisms within the ecosystem. Identification and prioritization of specific regions to be conserved are impossible without proper information about the sites. Advanced monitoring agencies like the Intergovernmental Science-Policy Platform on Biodiversity and Ecosystem Services (IPBES) had accredited that the sum total of species that are now threatened with extinction is higher than ever before in the past and are progressing toward extinct at an alarming rate. Besides this, the conceptualized global responses to these crises are still inadequate and entail drastic changes. Therefore, more sophisticated monitoring and conservation techniques are required which can simultaneously cover a larger surface area within a stipulated time frame and gather a large pool of data. Hence, this study is an overview of remote monitoring methods in biodiversity conservation via a survey of evidence-based reviews and related studies, wherein the description of the application of some technology for biodiversity conservation and monitoring is highlighted. Finally, the paper also describes various transformative smart technologies like artificial intelligence (AI) and/or machine learning algorithms for enhanced working efficiency of currently available techniques that will aid remote monitoring methods in biodiversity conservation.
Similar content being viewed by others
Remote sensing for biodiversity monitoring: a review of methods for biodiversity indicator extraction and assessment of progress towards international targets
Article 26 June 2015
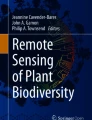
The Use of Remote Sensing to Enhance Biodiversity Monitoring and Detection: A Critical Challenge for the Twenty-First Century
Chapter © 2020
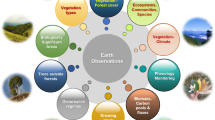
Remote sensing of biodiversity: what to measure and monitor from space to species?
Article 07 June 2021
Explore related subjects
- Artificial Intelligence
- Environmental Chemistry
Avoid common mistakes on your manuscript.
Introduction
Biological diversity “biodiversity” entails the assortment of earthly life forms heterogeneously ranging from genetic to ecosystem level. It can embrace the evolutionary, ecological, and cultural aspects that uphold life in various forms (McQuatters-Gollop et al. 2019). It fosters ecological functioning that paves the path for fundamental ecosystem services comprising food, water, preservation of soil fertility, and management of pests and diseases (Avigliano et al. 2019; Whitehorn et al. 2019). The plasticity of co-existence between mankind and nature is irreversible because of the symbiotic relationship that sustains the co-survival of humans with other living organisms (Arias-Maldonado 2016).
Biological diversity of forest originating from gene to ecosystem, through species, supports forest habitat that gives rise to fodders and other goods and services in a wide array of diverse biophysical and socio-economic ambience. Despite the applicability and significance of biodiversity, its conservation is vaguely acknowledged. Presently, human invasions have distorted around 75% of the land-based territory and about 66% of the marine ecosystem. Further to this, over a third of the global terrestrial regions are now devoted to domestic pursuit (FAO 2019). Moreover, since 1970, the significance of agricultural crop yield has increased by about 300%, and harvesting of raw timber has hiked by 45%. Moreover, renewable and non-renewable resources roughly of 60 billion tons are presently extracted annually across the globe. Exploitation of land has abridged the prolificacy of 23% of the global land area, annually, up to US$577 billion in worldwide crops are in jeopardy from pollinator loss, and about 100–300 million people are at elevated threat of natural disaster due to loss of coastal habitats and protection (IPBES 2019). If such trends continue then by 2050, the transformative change in nature can lead to an unprecedented devastating irreversible impact on mankind, which will take centuries to recover.
These atrocities of biodiversity need to be averted through proper monitoring and conservation measures. Based on the present advancements in technology, a combination of system-based smart techniques, remote sensing, and molecular approaches will be necessary for implementation of such ambitious conservation drives. Computer-based simulation techniques such as geographic information system (GIS), active and passive radio detection and ranging (RADAR) system, and light detection and ranging (LiDAR) system are playing a crucial role for monitoring biodiversity in real time (Bouvier et al. 2017; Bae et al. 2019; Bakx et al. 2019). Further to this, the application of recent advancements like artificial intelligence (AI) (Kwok 2019) and/or machine learning algorithms (Fernandes et al. 2020) have also been exploited for the same (Hu et al. 2015). These systems are not only reliable in monitoring biodiversity globally but can also help prevent further biodiversity loss worldwide. Besides monitoring tools, conservation of individual species and genetic biodiversity as a whole will require the use of recent molecular techniques. Conservation genomics revolves around the concept that genome-scale data will meliorate the competence of resource proprietors to conserve species. Despite the decades-long utilization of genetic approaches for conservation research, it has only recently been implied for generating genome-wide data which is functional for conservation (Supple and Shapiro 2018). The revolutionary molecular tools like restriction fragment length polymorphism (RFLP), amplified fragment length polymorphism (AFLP), random amplified polymorphic DNA (RAPD), sequence characterized amplified region (SCAR), microsatellites and mini-satellites, expressed sequence tags (ESTs), inter-simple sequence repeat (ISSR), and single nucleotide polymorphisms (SNPs) have transformed the hierarchy of biodiversity conservation to a higher level (Mosa et al. 2019).
Evidently, more sophisticated monitoring methods such as system-based simulation techniques, remote sensing, artificial intelligence, and geographic information system as well as molecular-based techniques facilitate the monitoring methods in biodiversity conservation and restoration. Therefore, the present study is an overview of remote monitoring methods in biodiversity conservation via a survey of evidence-based reviews and related studies, wherein the description of the application of some technology for biodiversity conservation and monitoring. Finally, the paper also describes various transformative smart technologies like artificial intelligence (AI) and/or machine learning algorithms for enhanced working efficiency of currently available techniques that will aid remote monitoring methods in biodiversity conservation.
Methodology of literature search
The relevant literature search was done electronically by using Google Search Engine, PubMed, ScienceDirect, SpringerLink, Frontiers Media, and MDPI databases. The most importantly searched keywords were biodiversity and potential threats, techniques to monitoring biodiversity, geographic information system, remote sensing, active remote sensing system, radio detection and ranging (RADAR) system, light detection and ranging (LiDAR) systems, passive remote sensing systems, techniques for identification and genetic conservation of species, restriction fragment length polymorphism (RFLP), amplified fragment length polymorphism (AFLP), random amplified polymorphic DNA (RAPD), sequence characterized amplified region (SCAR), mini- and micro-satellites, expressed sequence tags (ESTs), inter-simple sequence repeat (ISSR), single nucleotide polymorphisms (SNPs), and artificial intelligence in biodiversity monitoring which were used and placed repeatedly within the text.
Biodiversity and potential threats
Biodiversity in simple terms is a heterogenic distribution of flora and fauna throughout the world or in a particular niche (Naeem et al. 2016). The number of species described around the world as per IUCN (2020) accounts for 2,137,939, of which 72,327 are vertebrates, 1,501,581 are invertebrates, 422,756 are plants, and 141,275 are identified as fungi and protists. It is now acknowledged that biodiversity is a major indicator of community ecosystem fluctuations and functioning (Tilman et al. 2014). These include provisioning of food, pollination, cultural recreation, and supporting nutrient cycling (Harrison et al. 2014; Bartkowski et al. 2015). Biodiversity as a whole is represented by two major components that are species richness and species evenness. A biogeographic region with a significant level of endemic species and with a higher loss of habitat is generally depicted as a biodiversity hotspot (Marchese 2015). These areas have proven themselves as a tool for establishing conservation priorities and orchestrate vital rationale in decision-making for cost-effective tactics to safeguard biodiversity in its natural conserved state. Usually, the hotspots are marked by single or multiple species-based metrics or concentrate on phylogenetic and functional diversity to shield species that sustain exclusive and inimitable functions inside the ecosystem (Marchese 2015).
Currently, as per the IUCN Red List of Species 2020–2021, of the 2,137,939 species around the world, about 31,030 species are categorized as “threatened” species. Among these, plants with 16,460 numbers contribute the most followed by vertebrates (9063), invertebrates (5333), and fungi and protists (174) [IUCN 2020]. Many of the species are still not assessed due to a lack of reliable identification tools or techniques. Biodiversity is mostly threatened by over-population, habitat and landscape modification, indiscriminate exploitation of resources, pollution, and lack of proper documentation (Marchese 2015; Liu et al. 2020; Reid et al. 2019). Demographic changes can be considered an imperative module for assisting the indirect drivers of biodiversity alternations specifically associated with land use patterns (Newbold et al. 2015). Population explosion, central demographic developments, and urbanization impact both ecosystems and the species it harbors (Mehring et al. 2020). As the changing demographic pattern is associated with population explosion, this may pose a pessimistic impact on food availability, restricted emission of greenhouse gases, control of invasive species and diseases, etc. (Lampert 2019; Manisalidis et al. 2020; Hoban et al. 2020; Reid et al. 2019). To generate such massive data over a stipulated time frame and process them simultaneously to extrude applicable information requires cutting-edge tools and multidisciplinary scientific input (Randin et al. 2020). With recent advancements in mapping software, large-scale data processors, and monitoring tools and genetics, artificial intelligence for generating accurate data over a larger area as a part of a global monitoring strategy has now become feasible (Wetzel et al. 2015; Randin et al. 2020). Hence, an assortment of the above mention techniques and tools will be essential for the conservation and restoration of biodiversity.
Techniques for monitoring biodiversity
Mapping and monitoring techniques have been frontiers in predicting and modeling anthropogenic activities, habitat use, and pattern of land use over time in a particular region. These advanced physical techniques include GIS, LiDAR, and RADAR systems (Bouvier et al. 2017; Bae et al. 2019; Bakx et al. 2019).
Geographic information system (GIS)
Understanding functional geography and making intelligent decisions is widely beneficial for naturalists. GIS is a popular tool for analyzing possible and current spatial-temporal distribution, location, distribution patterns, population assessment, and identification of priority areas for their conservation and management (Krigas et al. 2012; Salehi and Ahmadian 2017). Currently, development of ecological niche models based on topographic, bioclimatic, soil, and land use variables was mapped and predicted for species such as Clinopodium nepeta, Thymbra capitata, Melissa officinalis, Micromeria juliana, Origanum dictamnus, O. vulgare, O. onites, Salvia fruticosa, S. pomifera, and Satureja thymbra (Bariotakis et al. 2019). With the assistance of digitally integrated video and audio-GIS (DIVA-GIS), actual geographic distribution and the future potential assortments of several Zingiber sp. like Z. mioga, Z. officinale, Z. striolatum, and Z. cochleariforme were analyzed (Huang et al. 2019). Most recently, important climatic inconsistencies distressing the geographical dispersion of wild Akebia trifoliate based on the formation of spatial database were successfully determined with the help of GIS (Wang et al. 2020). However, GIS possesses certain limitations such as expensive software, hardware, capturing GIS data, and difficulty in their use (Bearman et al. 2016) (Fig. 1, Table 1).
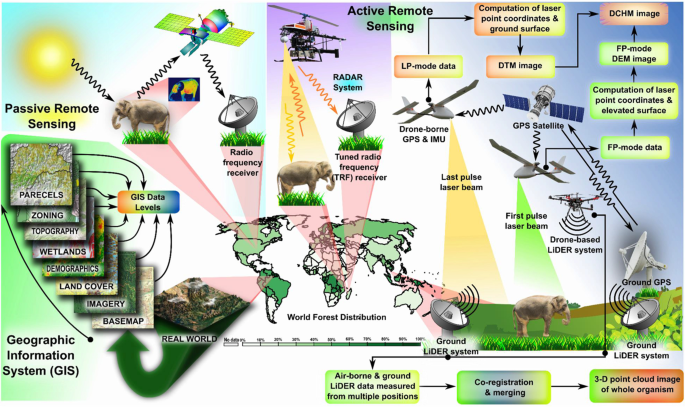
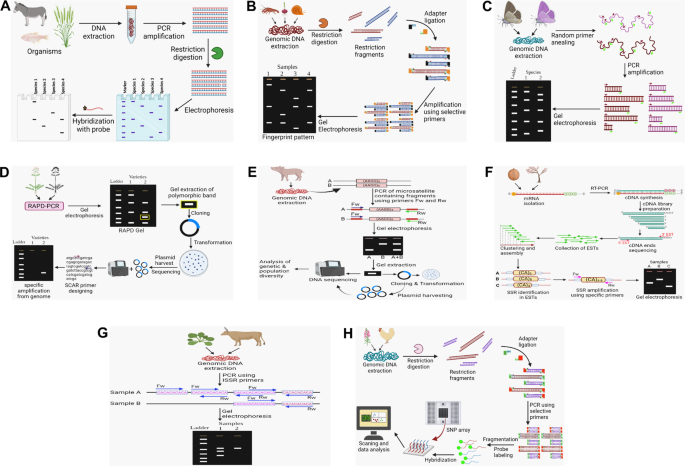
Amplified fragment length polymorphism (AFLP)
AFLP is considered an effective means of detecting polymorphism in DNA without having any prior information regarding the genome. Being a dominant marker, it can analyze multiple loci through amplification of DNA performing PCR reaction (Bryan et al. 2017). The method employs restriction digestion of DNA and amplification of fragments through ligation of adapters on both ends and using primers specific to adapters (Malik et al. 2018). Genetic differences can be identified from the disparity in the number and length of bands on electrophoretic separation. Its application ranges from the assessment of genetic diversity within species to generate of genetic maps for disease diagnosis and phylogenetic studies. AFLP data analysis study represents the genetic diversity in E. tangutorum population contributed by geographical and environmental factors (Wu et al. 2019b). The phylogenetic relationships and genetic distances among A. platensis populations and other distinct related species such as A. georginae and A. ludwigi in southern Brazil were also investigated through AFLP (Zimmermann et al. 2019). Population structure and differentiation among Melanopsis etrusca were clearly distinct between the eastern, western, and central regions populations in Italy (Neiber et al. 2020) (Table 3, Fig. 2B).
Random amplified polymorphic DNA (RAPD)
The RAPD is a PCR-based technique in which 8–10 short nucleotides comprise both forward and reverse primers that bind arbitrary nucleotide sequences of chromosomal DNA to generate random fragments. Due to this random nature of primers, no prior knowledge about genome sequence is needed. The annealing sites of these random primers vary for different species or individual to individual. Discrimination can be identified or determined from the amplified DNA fragments (RAPD markers) separated by agarose gel electrophoresis (Freigoun et al. 2020). RAPD markers are dominant and involved in various applications such as genome mapping, molecular evolutionary genetics, genetic diversity analysis, and population genetics as well as determining taxonomic identity (Qamer et al. 2021). Saikia et al. (2019) deduced genetic variation among the different morphs of muga silkworm of Northeast India through RAPD analysis. Moreover, Sulistyahadi et al. (2020) studied the locus diversity as well as genetic polymorphism of the endemic species Rhacophorus margaritifer population by this technique. It has also been used to elucidate the genetic variation in a medicinal plant species found in the south of Jordan named Artemisia judaica (Al-Rawashdeh 2011) (Table 3, Fig. 2C).
Sequence characterized amplified region (SCAR)
SCAR markers are DNA fragments generated by PCR amplification using specific 15–30-bp long primers derived from RAPD markers through cloning and sequencing (Bhagyawant 2015). Usually, RAPD markers are associated with low reproducibility and are dominant in nature, making it inappropriate for species identification (Sairkar et al. 2016). To overcome this disadvantage, RAPD markers are converted to SCAR markers which are locus-specific and co-dominant in nature (Bhagyawant 2015; Feng et al. 2018). Due to the specificity of primers, PCR amplification of SCARs is less sensitive to reaction condition and thus are easy to perform (Yuskianti and Shiraishi 2010). SCAR markers provide authenticate information both for species identification and population genetic diversity analysis. Researchers have successfully developed SCAR markers for the medicinal plant V. serpens using 1135-bp long amplicon through RAPD obtained by six accessions of the plant, thereby preventing it from extinction (Jha et al. 2020) (Table 3, Fig. 2D).
Mini- and micro-satellites
Mini-satellites (variable number of tandem repeats (VNTRs) 6–100 bp) and micro-satellites (1–6 bp) (simple sequence repeats (SSR) and short tandem repeats (STR)) are randomly repetitive DNA sequences widely dispersed in all eukaryotic species genomes. These multi-allelic markers are co-dominantly inherited with species-specific location and size within the genome (Vergnaud and Denoeud 2000; Vieira et al. 2016). Due to the high level of polymorphism associated with mini and microsatellites, it is extensively utilized in genetic analysis and population studies. Microsatellites are interspersed all over the genome and therefore represent high variability and their identification show great variation among species of the different population (Abdul-Muneer 2014). Its analysis includes PCR amplification of loci by using primers that flank the repeated sequence. By using microsatellite markers, genetic structure of Agu pigs has been elucidated along with its correlation with Ryukyu wild boar, two Chinese breeds and five European breeds (Touma et al. 2019). Similarly, De Góes Maciel et al. (2019) analyzed 13 microsatellite loci of 361 white-lipped peccaries for assessment of their population structure and level of genetic diversity (Table 3, Fig. 2E).
Expressed sequence tags (ESTs)
ESTs are small sequences of DNA usually 200 to 500 nucleotides long that act as tags for the expressed genes in certain cells, tissues, or organs. ESTs are generated by sequencing either the 3′ end or 5′ end of a segment derived from random clones from the cDNA library and long enough for the identity illustration of the expressed gene (Behera et al. 2013). ESTs are widely involved in gene discovery, determining the phylogenetic relationship between individuals, genetic diversity, and proteomic analysis as well as transcriptome profiling (Cai et al. 2015). EST-derived SSR markers are more informative than genomic SSRs for genetic diversity analysis due to several advantages such as high conserved nature, variation in coding sequence, and high heritability to closely related species (Parthiban et al. 2018). Sun et al. (2019) have conducted the structure analyses of expressed sequence tag-simple sequence repeat (EST-SSR) markers in Juglans sigillata and demonstrated the genetic structure based on its geographic feature. Moreover, EST-SSR analyses have provided information regarding the genetic distance between the J. regia and J. sigillata populations. By considering EST-SSRs and genotype sequencing data, they have interpreted iron walnut as the subspecies of J. regia (Sun et al. 2019). Investigation of evolutionary relations and genotypic relatedness are essential for the conservation of endangered species. Recently the genetic variability of an endangered species Magnolia patungensis was studied by analyzing the EST-SSR polymorphic markers (Wagutu et al. 2020) (Table 3, Fig. 2F).
Inter-simple sequence repeat (ISSR)
ISSR markers are used in diversified analyses such as species identification, evolutionary and taxonomic studies, genome mapping, genetic diversity, and gene tagging because of their high polymorphic nature (Arif et al. 2011; Abdelaziz et al. 2020). These multilocus markers are generated through PCR amplification by using microsatellites as primers. Prior sequence knowledge is not required for primer designing as repeat sequence is used to amplify these inter-microsatellite regions (Ng and Tan 2015). It overcomes all the limitations possessed by other markers such as RAPD and AFLP which are associated with low reproducibility (Najafzadeh et al. 2014). Genetic diversity and population structure analysis have been performed among 11 populations of Bergenia ciliata using 15 ISSR markers. The analysis shows a high level of polymorphism among this medicinal plant species, found in the Indian Himalayan Region (Tiwari et al. 2020). El Hentati et al. (2019) have studied genetic diversity and phylogenetic relationships among 20 samples of three geographical local cattle populations using ISSR primers. They found a significant variation and geographical separation among the cattle from the north, northeast, and northwest of Tunisia (Table 3, Fig. 2G).
Single nucleotide polymorphisms (SNPs)
Single nucleotide variation in genetic sequences defines the Single nucleotide polymorphism (SNP) among individuals, generated due to point mutation or replication errors, giving rise to different alleles within a locus (Van den Broeck et al. 2014). SNPs are the most common form of variation present extensively in the non-coding, coding, and inter-genic regions of DNA (Vallejos-Vidal et al. 2019). SNPs are mainly exploited for population structure, genetic diversity, genetic map construction, and identification of particular traits, etc. (Xia et al. 2019). Their abundance in coding regions makes them more attractive markers for the detection of mutations associated with diseases. SNP markers are however less polymorphic than SSR markers due to their biallelic or triallelic nature (Casci 2010; Mammadov et al. 2012). Cendron et al. (2020) demonstrated the population structure and genetic diversity of local Italian chicken breeds by using SNPs for conservation purposes which revealed lower genetic diversity among the local breeds. In another study, genetic diversity and differentiation among the D. ruyschiana populations of the Norwegian region were investigated by analyzing 96 SNPs derived from 43 sites that reported the existence of four distinct genetic groups within the population (Kyrkjeeide et al. 2020) (Table 3, Fig. 2H).
Artificial intelligence in biodiversity monitoring
With the growing performance of computing power and DL in recent years, machines had become significantly more intelligent and reliable than ever. Modern machines can handle more extensive data and more complex DL models than before (Dean 2019; Chen et al. 2020a). Through this progress, machines had achieved the ability to replicate human expertise (Liu et al. 2019b). Currently, several problems exist within our diverse planet. Researchers began to accelerate the development of several AI solutions with DL to preserve the earth for the later generations to come. In most studies, DL method’s employment provided an automated capability for machines to recognize, classify, and detect images, sounds, and behavior of animals, plants, and even humans (Abeßer 2020). According to Klein et al. (2015), one of the primary methods of preserving our biodiversity consists of monitoring and manual data collection. However, frequent conduct of such practices can become tedious and cause disturbances to sensitive wildlife habitats. With that said, monitoring became less reliable and brief (Table 4). AI-based methods have shown that even at its pre-mature level, biodiversity can have an improvement by reducing animal extinction, prolonged and in-depth monitoring of various life forms, unlocking and accessing unexplored areas, and faster and easier classification of species. With the continuing efforts in data duration, transparency, and research collaborations, these technology types may reach far beyond our expectations. These solutions, if appropriately handled, can yield a massive impact to preserve the planet and its resources without involving humans. Furthermore, the implementation of AI-based methods also extends humans’ capability to explore locations that our biological composition cannot handle, leading to discoveries of new species and life. Due to the accessibility of various capture devices, a wide range of collected data through images, videos, audio, and other forms of data fast-tracked DL and AI development. The problematic method and reliance on organic experts to perform a small to large-scale monitoring of animals, plants, and insects became less challenging as automation systems have improved significantly over a short period (Bergslien 2013; Buxton et al. 2018; Willi et al. 2018) (Fig. 3).
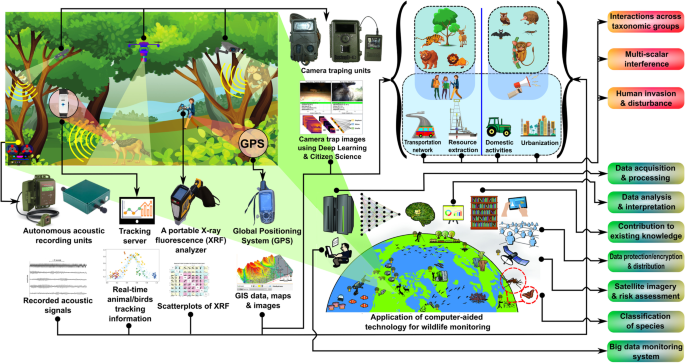
Recently, a wide range of low-cost yet powerful sensors, microphones, and cameras have become available, giving aid to alleviating the problem of collecting data. Such extensive data collections from the said technologies fueled DL models to learn more patterns that generated solutions to better monitor and manage biodiversity. The common uses include automated recognition, classification, and detection of people (Kim and Moon 2016), animals (Verma and Gupta 2018), plants (Saleem et al. 2019), fish (Jalal et al. 2020), and even insects (Xia et al. 2018) based on their sound or image (Christin et al. 2019). Even with DL’s promising capabilities, it still exhibits some caveats that limit its full potential in biodiversity monitoring, specifically in real time. Monitoring wildlife through video became an exponential and popular recent development that improved interpretability with less comprehension to researchers and the like (Chen et al. 2019). However, it became difficult and expensive due to the challenging deployment of capable computers or capture devices to perform the task (Willi et al. 2019). While operating with DL models in urban areas is relatively easy due to the availability of sufficient data on infrastructure, functioning in remote areas still relies on post-monitoring systems (He et al. 2016; Zhang et al. 2019a).
Researchers are also on for finding more efficient data collection techniques that will require less computational cost and fewer complexes. Currently, the computer on a hardware basis still rigorously improves and becomes more affordable and independently deployable. With that said, DL can become more efficient and reliable over time that can produce real-time wildlife monitoring in remote areas through a more visual aspect like videos without much constraint from the limited infrastructure.
Challenges and future prospects
Approximately, 1 million of the 10 million species that exist in the world are threatened with extinction (Bawa et al. 2020). Besides monitoring tools, a combination of efforts from varied disciplines will be essential for the safeguard of individual species and biodiversity as a whole. Computer model-based technologies like the GIS, RADAR, remote sensing, and LiDAR are actively used for the monitoring of habitats, state of threats, land uses, and conversion. Molecular approaches such as Mitochondrial DNA (Cyt b), SNPs, RFLP, microsatellites, etc. are also playing a pivotal role in identifying, tracking, and determining the impact of anthropogenic and environmental factors on wildlife (Krestoff et al. 2021; Gouda et al. 2020; Ridley et al. 2020). However, many of these techniques face challenges in form of cost-efficiency and expert handling and have single or limited focal species at the ecosystem level. Some of the possible changes and prospects in biodiversity monitoring systems that can be implemented in near future on broader aspects are discussed below.
The science of chorology with advances in GIS and remote sensing techniques in recent times has better presented the landscape as a functional unit for biodiversity management. Visualizations of spatial-temporal changes and development of biotic and abiotic threats to species also known as “threat maps” emerged as multipurpose techniques for the implementation of conservation activities at the ground level (Ridley et al. 2020). InVEST (Integrated Valuation of Ecosystem Services and Trade-offs) is a newly develop modeling software with set parameters for screening and quantification of ecosystem services such as carbon stock, changes in land use, landscape, forest cover, etc. SolVES is a modern-day ArcGIS-dependent tool that provides the user with easy access to several functions of the Ecosystem Services (ES), human perceptions associated with social and cultural beliefs, socio-economic values, usage of resources, etc. even without conducting questionnaires or other ground surveys of the local people and other stakeholders (Neugarten et al. 2020).
ARIES (ARtificial Intelligence for Ecosystem Services) is a series of algorithm processes which are generated through detecting or recognizing and keeping the track of living systems. It is a software-based platform that solves complex and arduous social or bio-geographical dimensions by integrating biodiversity data (Silvestro et al. 2022). It has been successfully tested for carbon emission, climate change, water levels, and ethnic/recreational values (Bagstad et al. 2018). Costing Nature is another easy-to-use rapid and reliable web-based technique used for screening protected areas, land use and land cover (LULC), trends of habitation, biodiversity assessment, and possible future threats using global database. It has been used for testing ES for timber, fuel wood, grazing/fodder, and non-wood forest products (Thessen 2016; Dominguez-Morales et al. 2021; Neugarten et al. 2018).
As rightly pointed out by Malavasi (2020), biodiversity maps are always selective and do not necessarily display all values that are known about any given region or ecosystem. They are often inevitably affected by personal views or scientific blindness and it is therefore important to strive and rate maps not only in terms of scientific accuracy but also on their “viability.” The use of Public Participation Geographic Information Systems (PPGIS) over conventional screening systems can act as a bottom-up approach to empower concern agencies about the threats and conservation priorities by providing visual tools. Similarly, the use of a counter map can prove as a possible substitute for mitigating the loss of biodiversity in a more “systemic” manner (Schägner et al. 2013; Malavasi 2020).
Genomics models and concepts are widely applied for biodiversity sustenance, from ideal seed selection for preservation to assessing the degree of impact at community-level effects. The concept of population genomics has provided valuable information on population size, demographic history, ability of the populations to evolve and adapt to the changing environment, etc. (Miraldo et al. 2016; Hu et al. 2020, 2021; Hohenlohe et al. 2021). They have been able to successfully develop large sets of markers that increase the ability to detect and quantify low levels of hybridization or admixture. Techniques such as intron sequences with assistance from Transcriptome Ortholog Alignment Sequence Tools (TOASTs), Next-Generation Sequencing (NGS), and Comparative Anchor Tagged Sequences (CATs) may represent a good proxy to assess functional adaptive potential or functional diversity in future genomic studies (Forcina et al. 2021).
Conclusion
With continuous advances in technology, more precise and reliable techniques have been designed for biodiversity conservation. However, association mapping and expanding knowledge on “omics” will help in identifying morphological traits and bring together intellectual minds to a platform for developing advanced gene traits. It also helps identify high biodiversity conservation priority areas or hotspots. Working closely with international agencies like the Convention on Biological Diversity (CBD) and UN Framework Convention on Climate Change (UNFCCC) and achieving its targets will be important for the conservation of biodiversity on the planet. Lastly, it is the human who understands the importance of coexistence and cohabitation with other forms of living beings that will help implement conservation measures and create a sense of protecting the ecosystem. Therefore, it is suggested that a combination of sophisticated monitoring methods including system-based smart techniques, transformative smart technologies, remote sensing, geographical information system, and artificial intelligence in combination with molecular approaches will smartly keep the track of living organisms and will help in biodiversity conservation and restoration.
Availability of data and materials
All data generated or analyzed during this study are included in this article.
References
- Abdelaziz SM, Medraoui L, Alami M, Pakhrou O, Makkaoui M, Boukhary OMS, Filali-Maltouf A (2020) Inter simple sequence repeat markers to assess genetic diversity of the desert date (Balanites aegyptiaca Del.) for Sahelian ecosystem restoration. Sci Rep 10:14948
- Abdul-Muneer PM (2014) Application of microsatellite markers in conservation genetics and fisheries management: recent advances in population structure analysis and conservation strategies. Gen Res Int 2014:691759 CASGoogle Scholar
- Abeßer J (2020) A review of deep learning based methods for acoustic scene classification. Appl Sci 10:2020 ArticleGoogle Scholar
- Ade FY, Hakim L, Arumingtyas EL, Azrianingsih R (2019) The detection of Anaphalis spp. genetic diversity based on molecular character (using ITS, ETS, and EST-SSR markers). Int J Adv Sci Eng Inform Technol 9:1695–1702 ArticleGoogle Scholar
- Admin (2017) My blog, GIS Layers, Environmental Science and Resource Management. http://heleneloyan.cikeys.com/update/gis-layers/. Accessed 11 Aug 2021
- Akçay HG, Kabasakal B, Aksu D, Demir N, Öz M, Erdoğan A (2020) Automated bird counting with deep learning for regional bird distribution mapping. Animals : an Open Access Journal from MDPI 10:1207 ArticleGoogle Scholar
- Al-Allak ZS, Dragh MA, Hussain AS (2020) Genetic polymorphism and diversity of Iraqi Awassi sheep using PCR-RAPD technique. Basrah J Vet Res 19:147–154 ArticleGoogle Scholar
- Alemu A, Feyissa T, Letta T, Abeyo B (2020) Genetic diversity and population structure analysis based on the high density SNP markers in Ethiopian durum wheat (Triticum turgidum ssp. durum). BMC Genet 21:18 ArticleCASGoogle Scholar
- Alexander C, Korstjens AH, Usher G, Nowak MG, Fredriksson G, Hill RA (2018) LiDAR patch metrics for object-based clustering of forest types in a tropical rainforest. Int J Appl Earth Obs Geoinf 73:253–261 Google Scholar
- Ali AM, Darvishzadeh R, Skidmore A, Gara TW, Heurich M (2021) Machine learning methods’ performance in radiative transfer model inversion to retrieve plant traits from Sentinel-2 data of a mixed mountain forest. Int J Digital Earth 14:106–120 ArticleGoogle Scholar
- Almeida DRA, Broadbent EN, Zambrano AMA, Wilkinson BE, Ferreira ME, Chazdon R, Meli P, Gorgens EB, Silva CA, Stark SC, Valbuena R, Papa DA, Brancalion PHS (2019) Monitoring the structure of forest restoration plantations with a drone-lidar system. Int J Appl Earth Obs Geoinf 79:192–198 Google Scholar
- Al-Rawashdeh IM (2011) Genetic variability in a medicinal plant Artemisia judaica using random amplified polymorphic DNA (RAPD) markers. Int J Agr Biol 13:279–282 CASGoogle Scholar
- Alsolami R, Knight SJ, Schuh A (2013) Clinical application of targeted and genome-wide technologies: can we predict treatment responses in chronic lymphocytic leukemia? Person Med 10:361–376 ArticleCASGoogle Scholar
- Amom T, Nongdam P (2017) The use of molecular marker methods in plants: a review. Int J Curr Res Rev 9:01–07 CASGoogle Scholar
- Arias-Maldonado M (2016) The anthropocenic turn: theorizing sustainability in a postnatural age. Sustainability 8:10 ArticleGoogle Scholar
- Arif IA, Khan HA, Bahkali AH, Al Homaidan AA, Al Farhan AH, Al Sadoon M, Shobrak M (2011) DNA marker technology for wildlife conservation. Saudi J Biol Sci 18:219–225 ArticleCASGoogle Scholar
- Arshad B, Barthelemy J, Pilton E, Perez P (2020) Where is my deer?-wildlife tracking and counting via edge computing and deep learning. In: 2020 IEEE SENSORS. IEEE, Rotterdam, Netherlands, pp 1–4
- Avigliano E, Rosso JJ, Lijtmaer D, Ondarza P, Piacentini L, Izquierdo M, Cirigliano A, Romano G, Nuñez Bustos E, Porta A, Mabragaña E, Grassi E, Palermo J, Bukowski B, Tubaro P, Schenone N (2019) Biodiversity and threats in non-protected areas: a multidisciplinary and multi-taxa approach focused on the Atlantic Forest. Heliyon 5:e02292 ArticleGoogle Scholar
- Bae S, Levick SR, Heidrich L, Magdon P, Leutner BF, Wöllauer S, Serebryanyk A, Nauss T, Krzystek P, Gossner MM, Schall P, Heibl C, Bässler C, Doerfler I, Schulze E-D, Krah F-S, Culmsee H, Jung K, Heurich M et al (2019) Radar vision in the mapping of forest biodiversity from space. Nat Commun 10:4757 ArticleGoogle Scholar
- Bagstad KJ, Cohen E, Ancona ZH, McNulty SG, Sun G (2018) The sensitivity of ecosystem service models to choices of input data and spatial resolution. Appl Geogr 93:25–36 ArticleGoogle Scholar
- Bakx TRM, Koma Z, Seijmonsbergen AC, Kissling WD (2019) Use and categorization of light detection and ranging vegetation metrics in avian diversity and species distribution research. Divers Distrib 25:1045–1059 ArticleGoogle Scholar
- Bariotakis M, Georgescu L, Laina D, Oikonomou I, Ntagounakis G, Koufaki M-I, Souma M, Choreftakis M, Zormpa OG, Smykal P, Sourvinos G, Lionis C, Castanas E, Karousou R, Pirintsos SA (2019) From wild harvest towards precision agriculture: use of ecological niche modelling to direct potential cultivation of wild medicinal plants in Crete. Sci Total Environ 694:133681 ArticleCASGoogle Scholar
- Barlow SE, O’Neill MA (2020) Technological advances in field studies of pollinator ecology and the future of e-ecology. Curr Opin Insect Sci 38:15–25 ArticleGoogle Scholar
- Bartkowski B, Lienhoop N, Hansjürgens B (2015) Capturing the complexity of biodiversity: a critical review of economic valuation studies of biological diversity. Ecol Econ 113:1–14 ArticleGoogle Scholar
- Basak S, Chakrabartty I, Hedaoo V, Shelke RG, Rangan L (2019) Assessment of genetic variation among wild Alpinia nigra (Zingiberaceae) population: an approach based on molecular phylogeny. Mol Biol Rep 46:177–189 ArticleCASGoogle Scholar
- Baumann M, Levers C, Macchi L, Bluhm H, Waske B, Gasparri NI, Kuemmerle T (2018) Mapping continuous fields of tree and shrub cover across the Gran Chaco using Landsat 8 and Sentinel-1 data. Remote Sens Environ 216:201–211 ArticleGoogle Scholar
- Bawa KS, Nawn N, Chellam R, Krishnaswamy J, Mathur V, Olsson SB, Pandit N, Rajagopal P, Sankaran M, Shaanker RU, Shankar D, Ramakrishnan U, Vanak AT, Quader S (2020) Opinion: envisioning a biodiversity science for sustaining human well-being. Proc Natl Acad Sci 117:25951–25955 ArticleCASGoogle Scholar
- Bearman N, Jones N, André I, Cachinho HA, DeMers M (2016) The future role of GIS education in creating critical spatial thinkers. J Geogr High Educ 40:394–408 ArticleGoogle Scholar
- Behera PM, Behera DK, Panda A, Dixit A, Padhi P (2013) In silico expressed sequence tag analysis in identification of probable diabetic genes as virtual therapeutic targets. Biomed Res Int 2013:704818 ArticleGoogle Scholar
- Belenguer-Plomer MA, Tanase MA, Fernandez-Carrillo A, Chuvieco E (2019) Burned area detection and mapping using Sentinel-1 backscatter coefficient and thermal anomalies. Remote Sens Environ 233:111345 ArticleGoogle Scholar
- Bergslien ET (2013) X-ray diffraction and field portable X-ray fluorescence analysis and screening of soils: project design. Geol Soc Lond, Spec Publ 384:27–46 ArticleCASGoogle Scholar
- Bhagyawant SS (2015) RAPD-SCAR Markers: an interface tool for authentication of traits. J Biosci Med 4:1–9 Google Scholar
- Bhatta NP, Priya MG (2017) Radar and its applications. Int J Control Theory Appl 10:1–9 Google Scholar
- Bispo PDC, Pardini M, Papathanassiou KP, Kugler F, Balzter H, Rains D, dos Santos JR, Rizaev IG, Tansey K, dos Santos MN, Spinelli Araujo L (2019) Mapping forest successional stages in the Brazilian Amazon using forest heights derived from TandEM-X SAR interferometry. Remote Sens Environ 232:111194 ArticleGoogle Scholar
- Bjerge K, Nielsen JB, Sepstrup MV, Helsing-Nielsen F, Høye TT (2021) An automated light trap to monitor moths (lepidoptera) using computer vision-based tracking and deep learning. Sensors 21:343 ArticleGoogle Scholar
- Blears MJ, De Grandis SA, Lee H, Trevors JT (1998) Amplified fragment length polymorphism (AFLP): a review of the procedure and its applications. J Ind Microbiol Biotechnol 21:99–114 ArticleCASGoogle Scholar
- Bolton DK, Tompalski P, Coops NC, White JC, Wulder MA, Hermosilla T, Queinnec M, Luther JE, van Lier OR, Fournier RA, Woods M, Treitz PM, van Ewijk KY, Graham G, Quist L (2020) Optimizing Landsat time series length for regional mapping of lidar-derived forest structure. Remote Sens Environ 239:111645 ArticleGoogle Scholar
- Bouvier M, Durrieu S, Gosselin F, Herpigny B (2017) Use of airborne lidar data to improve plant species richness and diversity monitoring in lowland and mountain forests. PLoS One 12:e0184524 ArticleGoogle Scholar
- Bowler E, Fretwell PT, French G, Mackiewicz M (2020) Using deep learning to count albatrosses from space: assessing results in light of ground truth uncertainty. Remote Sens 12:2026 ArticleGoogle Scholar
- Bryan GJ, McLean K, Waugh R, Spooner DM (2017) Levels of Intra-specific AFLP diversity in tuber-bearing potato species with different breeding systems and ploidy levels. Front Genet 8:119 ArticleGoogle Scholar
- Buss MEF, Leizica E, Peinetti R, Noellemeyer E (2020) Relationships between landscape features, soil properties, and vegetation determine ecological sites in a semiarid savanna of central Argentina. J Arid Environ 173:104038 ArticleGoogle Scholar
- Buxton RT, Lendrum PE, Crooks KR, Wittemyer G (2018) Pairing camera traps and acoustic recorders to monitor the ecological impact of human disturbance. Global Ecol Conserv 16:e00493 ArticleGoogle Scholar
- Cai C, Yang Y, Cheng L, Tong C, Feng J (2015) Development and assessment of EST-SSR marker for the genetic diversity among tobaccos (Nicotiana tabacum L.). Russ J Genet 51:591–600 ArticleCASGoogle Scholar
- Cao L, Coops NC, Sun Y, Ruan H, Wang G, Dai J, She G (2019) Estimating canopy structure and biomass in bamboo forests using airborne LiDAR data. ISPRS J Photogramm Remote Sens 148:114–129 ArticleGoogle Scholar
- Carr A, Zeale MRK, Weatherall A, Froidevaux JSP, Jones G (2018) Ground-based and LiDAR-derived measurements reveal scale-dependent selection of roost characteristics by the rare tree-dwelling bat Barbastella barbastellus. For Ecol Manag 417:237–246 ArticleGoogle Scholar
- Carreiras JMB, Jones J, Lucas RM, Shimabukuro YE (2017) Mapping major land cover types and retrieving the age of secondary forests in the Brazilian Amazon by combining single-date optical and radar remote sensing data. Remote Sens Environ 194:16–32 ArticleGoogle Scholar
- Casci T (2010) SNPs that come in threes. Nat Rev Genet 11:8–8 ArticleGoogle Scholar
- Cavender-Bares J, Gamon JA, Townsend PA (2020) The use of remote sensing to enhance biodiversity monitoring and detection: a critical challenge for the twenty-first century. In: Cavender-Bares J, Gamon JA, Townsend PA (eds) Remote sensing of plant biodiversity. Springer International Publishing, Cham, pp 1–12 ChapterGoogle Scholar
- Cendron F, Perini F, Mastrangelo S, Tolone M, Criscione A, Bordonaro S, Iaffaldano N, Castellini C, Marzoni M, Buccioni A, Soglia D, Schiavone A, Cerolini S, Lasagna E, Cassandro M (2020) Genome-wide SNP analysis reveals the population structure and the conservation status of 23 Italian chicken breeds. Animals : an Open Access Journal from MDPI 10:1441 ArticleGoogle Scholar
- Chang J, Shoshany M (2017) Radar polarization and ecological pattern properties across Mediterranean-to-arid transition zone. Remote Sens Environ 200:368–377 ArticleGoogle Scholar
- Chaudhary R, Maurya GK (2019) In: Vonk J, Shackelford T (eds) Restriction fragment length polymorphism. Encyclopedia of Animal Cognition and Behavior. Springer International Publishing, Cham, pp 1–3 Google Scholar
- Chen R, Little R, Mihaylova L, Delahay R, Cox R (2019) Wildlife surveillance using deep learning methods. Ecol Evol 9:9453–9466 ArticleGoogle Scholar
- Chen M-Y, Chiang H-S, Lughofer E, Egrioglu E (2020a) Deep learning: emerging trends, applications and research challenges. Soft Comput 24:7835–7838 ArticleGoogle Scholar
- Chen X, Zhao J, Chen Y, Zhou W, Hughes AC (2020b) Automatic standardized processing and identification of tropical bat calls using deep learning approaches. Biol Conserv 241:108269 ArticleGoogle Scholar
- Christin S, Hervet É, Lecomte N (2019) Applications for deep learning in ecology. Methods Ecol Evol 10:1632–1644 ArticleGoogle Scholar
- Chunming W, Guoliang D (2012) The study of UWB RADAR life-detection for searching human subjects. Energy Procedia 17:1028–1033 ArticleGoogle Scholar
- Clapham M, Miller E, Nguyen M, Darimont CT (2020) Automated facial recognition for wildlife that lack unique markings: a deep learning approach for brown bears. Ecol Evol 10:12883–12892 ArticleGoogle Scholar
- Crabbe RA, Lamb D, Edwards C (2020) Discrimination of species composition types of a grazed pasture landscape using Sentinel-1 and Sentinel-2 data. Int J Appl Earth Obs Geoinf 84:101978 Google Scholar
- Cunha JT, Domingues L (2017) RAPD/SCAR Approaches for identification of adulterant breeds’ milk in dairy products. Methods Mol Biol (Clifton NJ) 1620:183–193 ArticleCASGoogle Scholar
- Curry CJ, Davis BW, Bertola LD, White PA, Murphy WJ, Derr JN (2021) Spatiotemporal genetic diversity of lions reveals the influence of habitat fragmentation across Africa. Mol Biol Evol 38:48–57 ArticleCASGoogle Scholar
- Dalponte M, Jucker T, Liu S, Frizzera L, Gianelle D (2019) Characterizing forest carbon dynamics using multi-temporal lidar data. Remote Sens Environ 224:412–420 ArticleGoogle Scholar
- de Góes Maciel F, Rufo DA, Keuroghlian A, Russo AC, Brandt NM, Vieira NF, da Nóbrega BM, Nava A, Nardi MS, de Almeida Jácomo AT, Silveira L, Furtado MM, Tôrres NM, Miyaki CY, Tambosi LR, Biondo C (2019) Genetic diversity and population structure of white-lipped peccaries (Tayassu pecari) in the Pantanal, Cerrado and Atlantic Forest from Brazil. Mamm Biol 95:85–92 ArticleGoogle Scholar
- Dean J (2019) The deep learning revolution and its implications for computer architecture and chip design. http://arxiv.org/abs/1911.05289. Accessed 12 Aug 2021
- Ditria EM, Lopez-Marcano S, Sievers M, Jinks EL, Brown CJ, Connolly RM (2020) Automating the analysis of fish abundance using object detection: optimizing animal ecology with deep learning. Front Mar Sci 7:429 ArticleGoogle Scholar
- Dominguez-Morales JP, Duran-Lopez L, Gutierrez-Galan D, Rios-Navarro A, Linares-Barranco A, Jimenez-Fernandez A (2021) Wildlife monitoring on the edge: a performance evaluation of embedded neural networks on microcontrollers for animal behavior classification. Sensors 21:2975 ArticleGoogle Scholar
- Dube T, Shoko C, Sibanda M, Madileng P, Maluleke XG, Mokwatedi VR, Tibane L, Tshebesebe T (2020) Remote Sensing of Invasive Lantana camara (Verbenaceae) in Semiarid Savanna Rangeland Ecosystems of South Africa. Rangel Ecol Manag 73:411–419 ArticleGoogle Scholar
- Duporge I, Isupova O, Reece S, Macdonald DW, Wang T (2020) Using very-high-resolution satellite imagery and deep learning to detect and count African elephants in heterogeneous landscapes. Remote Sens Ecol Conserv 7(3):369–381 n/a ArticleGoogle Scholar
- Earthdata (2021) Remote Sensors. Earthdata.
- Ebrahimi R, Hassandokht MR, Zamani Z, Roldan-Ruiz I, Muylle H, Van Glabeke S, Van Bockstaele E, Kashi A (2019) Genetic characterization of Allium stipitatum accessions: an economically wild edible Allium species with unique flavor. Braz J Bot 42:83–96 ArticleGoogle Scholar
- El Hentati H, Thamri N, Derouich W, Hadhli M, Boukhorsa T (2019) Study of genetic diversity in Tunisian local cattle populations using ISSR markers. J Anim Plant Sci 42(3):7296–7302 ArticleGoogle Scholar
- El-Demerdash E-SS, Elsherbeny EA, Salama YAM, Ahmed MZ (2019) Genetic diversity analysis of some Egyptian origanum and Thymus species using AFLP markers. J Gen Eng Biotechnol 17:13 ArticleGoogle Scholar
- Erinjery JJ, Singh M, Kent R (2018) Mapping and assessment of vegetation types in the tropical rainforests of the Western Ghats using multispectral Sentinel-2 and SAR Sentinel-1 satellite imagery. Remote Sens Environ 216:345–354 ArticleGoogle Scholar
- Esmaeili H, Karami A, Hadian J, Nejad Ebrahimi S, Otto L-G (2020) Genetic structure and variation in Iranian licorice (Glycyrrhiza glabra L.) populations based on morphological, phytochemical and simple sequence repeats markers. Ind Crop Prod 145:112140 ArticleCASGoogle Scholar
- FAO, A (2019) The state of food and agriculture. 2019. In: 2019, Moving forward on food loss and waste reduction. Food and Agriculture Organization of the United Nations, Rome Google Scholar
- Fauvel M, Lopes M, Dubo T, Rivers-Moore J, Frison P-L, Gross N, Ouin A (2020) Prediction of plant diversity in grasslands using Sentinel-1 and -2 satellite image time series. Remote Sens Environ 237:111536 ArticleGoogle Scholar
- Fedrigo M, Newnham GJ, Coops NC, Culvenor DS, Bolton DK, Nitschke CR (2018) Predicting temperate forest stand types using only structural profiles from discrete return airborne lidar. ISPRS J Photogramm Remote Sens 136:106–119 ArticleGoogle Scholar
- Feng S, Zhu Y, Yu C, Jiao K, Jiang M, Lu J, Shen C, Ying Q, Wang H (2018) Development of species-specific SCAR markers, based on a SCoT analysis, to authenticate Physalis (Solanaceae) species. Front Genet 9:192 ArticleGoogle Scholar
- Fernandes ACM, Gonzalez RQ, Lenihan-Clarke MA, Trotter EFL, Arsanjani JJ (2020) Machine learning for conservation planning in a changing climate. Sustainability 12:7657 ArticleGoogle Scholar
- Fernandez-Carrillo A, McCaw L, Tanase MA (2019) Estimating prescribed fire impacts and post-fire tree survival in eucalyptus forests of Western Australia with L-band SAR data. Remote Sens Environ 224:133–144 ArticleGoogle Scholar
- Ferreira RC, Piredda R, Bagnoli F, Bellarosa R, Attimonelli M, Fineschi S, Schirone B, Simeone MC (2011) Phylogeography and conservation perspectives of an endangered macaronesian endemic: Picconia azorica (Tutin) Knobl. (Oleaceae). Eur J For Res 130:181–195 ArticleGoogle Scholar
- Forcina G, Camacho-Sanchez M, Tuh FYY, Moreno S, Leonard JA (2021) Markers for genetic change. Heliyon 7:e05583 ArticleCASGoogle Scholar
- Freepik (2021) Download 3d isometric terrain of a mountainous landscape for free. In: Freepik. href='https://www.freepik.com/photos/map'>Map photo created by kjpargeter - www.freepik.com. Accessed 9 Aug 2021
- Freigoun SAB, Elagib TY, Raddad EYA (2020) Analysis of genetic diversity in four Sudanese provenances of Balanites aegyptiaca (L.) Del. based on random amplified polymorphic DNA (RAPD) marker. Afr J Biotechnol 19:408–414 ArticleCASGoogle Scholar
- Gallardo-Alvárez MI, Lesher-Gordillo JM, Machkour-M’Rabet S, Zenteno-Ruiz CE, Olivera-Gómez LD, del Rosario Barragán-Vázquez M, Ríos-Rodas L, Valdés-Marín A, Vázquez-López HG, Arriaga-Weiss SL (2019) Genetic diversity and population structure of founders from wildlife conservation management units and wild populations of critically endangered Dermatemys mawii. Global Ecol Conserv 19:e00616 ArticleGoogle Scholar
- Gamal E, Khdery G, Morsy A, Ali M, Hashim A, Saleh H (2020) Using GIS based modelling to aid conservation of two endangered plant species (Ebenus armitagei and Periploca angustifolia) at Wadi Al-Afreet, Egypt. Remote Sens Appl: Soc Environ 19:100336 Google Scholar
- Ganie SH, Upadhyay P, Das S, Prasad Sharma M (2015) Authentication of medicinal plants by DNA markers. Plant Gene 4:83–99 ArticleCASGoogle Scholar
- García M, Saatchi S, Ustin S, Balzter H (2018) Modelling forest canopy height by integrating airborne LiDAR samples with satellite Radar and multispectral imagery. Int J Appl Earth Obs Geoinf 66:159–173 Google Scholar
- Ge Z, Dai Z, Pang W, Li S, Wei W, Mei X, Huang H, Gu J (2017) LIDAR-based detection of the post-typhoon recovery of a meso-macro-tidal beach in the Beibu Gulf, China. Mar Geol 391:127–143 ArticleGoogle Scholar
- Goncalves AL, García MV, Heuertz M, González-Martínez SC (2019) Demographic history and spatial genetic structure in a remnant population of the subtropical tree Anadenanthera colubrina var. cebil (Griseb.) Altschul (Fabaceae). Ann For Sci 76:18 ArticleGoogle Scholar
- González-Rivero M, Beijbom O, Rodriguez-Ramirez A, Bryant DEP, Ganase A, Gonzalez-Marrero Y, Herrera-Reveles A, Kennedy EV, Kim CJS, Lopez-Marcano S, Markey K, Neal BP, Osborne K, Reyes-Nivia C, Sampayo EM, Stolberg K, Taylor A, Vercelloni J, Wyatt M, Hoegh-Guldberg O (2020) Monitoring of coral reefs using artificial intelligence: a feasible and cost-effective approach. Remote Sens 12:489 ArticleGoogle Scholar
- Gouda S, Kerry RG, Das A, Chauhan NS (2020) Wildlife forensics: a boon for species identification and conservation implications. Forensic Sci Int 317:110530 ArticleCASGoogle Scholar
- Griffiths P, Nendel C, Pickert J, Hostert P (2020) Towards national-scale characterization of grassland use intensity from integrated Sentinel-2 and Landsat time series. Remote Sens Environ 238:111124 ArticleGoogle Scholar
- Große-Stoltenberg A, Hellmann C, Thiele J, Werner C, Oldeland J (2018) Early detection of GPP-related regime shifts after plant invasion by integrating imaging spectroscopy with airborne LiDAR. Remote Sens Environ 209:780–792 ArticleGoogle Scholar
- Guo X, Coops NC, Tompalski P, Nielsen SE, Bater CW, John Stadt J (2017) Regional mapping of vegetation structure for biodiversity monitoring using airborne lidar data. Ecol Inform 38:50–61 ArticleGoogle Scholar
- Guo Y, Liao J, Shen G (2021) Mapping large-scale mangroves along the maritime silk road from 1990 to 2015 using a novel deep learning model and landsat data. Remote Sens 13:245 ArticleGoogle Scholar
- Haas J, Ban Y (2017) Sentinel-1A SAR and sentinel-2A MSI data fusion for urban ecosystem service mapping. Remote Sens Appl: Soc Environ 8:41–53 Google Scholar
- Haider N, Nabulsi I (2020) Identification of bread and durum wheats from their diploid ancestral species based on chloroplast DNA. Agriculture (Pol’nohospodárstvo) 66:56–66 Google Scholar
- Hao J, Jiao K, Yu C, Guo H, Zhu Y, Yang X, Zhang S, Zhang L, Feng S, Song Y, Dong M, Wang H, Shen C (2018) Development of SCoT-based SCAR marker for rapid authentication of Taxus media. Biochem Genet 56:255–266 ArticleCASGoogle Scholar
- Harrison PA, Berry PM, Simpson G, Haslett JR, Blicharska M, Bucur M, Dunford R, Egoh B, Garcia-Llorente M, Geamănă N, Geertsema W, Lommelen E, Meiresonne L, Turkelboom F (2014) Linkages between biodiversity attributes and ecosystem services: a systematic review. Ecosyst Serv 9:191–203 ArticleGoogle Scholar
- Hay SI (2000) An overview of remote sensing and geodesy for epidemiology and public health application. Adv Parasitol 47:1–35 ArticleCASGoogle Scholar
- He Z, Kays R, Zhang Z, Ning G, Huang C, Han TX, Millspaugh J, Forrester T, McShea W (2016) Visual informatics tools for supporting large-scale collaborative wildlife monitoring with citizen scientists. IEEE Circuits SystMag 16:73–86 ArticleGoogle Scholar
- Hirst M (2008) Operational environment. The air transport system. Woodhead Publishing, Cambridge, pp 72–101 Google Scholar
- Hoban S, Bruford M, D’Urban Jackson J, Lopes-Fernandes M, Heuertz M, Hohenlohe PA, Paz-Vinas I, Sjögren-Gulve P, Segelbacher G, Vernesi C, Aitken S, Bertola LD, Bloomer P, Breed M, Rodríguez-Correa H, Funk WC, Grueber CE, Hunter ME, Jaffe R et al (2020) Genetic diversity targets and indicators in the CBD post-2020 Global biodiversity framework must be improved. Biol Conserv 248:108654 ArticleGoogle Scholar
- Hohenlohe PA, Funk WC, Rajora OP (2021) Population genomics for wildlife conservation and management. Mol Ecol 30:62–82 ArticleGoogle Scholar
- Höppler L, Gödde F, Gutleben M et al (2020) Synergy of active- and passive remote sensing: An approach to reconstruct three-dimensional cloud macro- and microphysics. https://www.atmos-meas-tech-discuss.net/amt-2020-49/. Accessed 12 Aug 2021
- Høye TT, Ärje J, Bjerge K, Hansen OLP, Iosifidis A, Leese F, Mann HMR, Meissner K, Melvad C, Raitoharju J (2021) Deep learning and computer vision will transform entomology. Proc Natl Acad Sci 118:e2002545117 ArticleGoogle Scholar
- Hu J, Rampitsch C, Bykova NV (2015) Advances in plant proteomics toward improvement of crop productivity and stress resistancex. Front Plant Sci 6:209 ArticleGoogle Scholar
- Hu C, Pan T, Wu Y, Zhang C, Chen W, Chang Q (2020) Spatial genetic structure and historical demography of East Asian wild boar. Anim Genet 51:557–567 ArticleCASGoogle Scholar
- Hu C, Yuan S, Sun W, Chen W, Liu W, Li P, Chang Q (2021) Spatial genetic structure and demographic history of the wild boar in the Qinling Mountains, China. Animals 11:346 ArticleGoogle Scholar
- Huang Z, Xie L, Wang H, Zhong J, Li Y, Liu J, Ou Z, Liang X, Li Y, Huang H, Lin Z, Zhang K, Zhang L, Zheng X (2019) Geographic distribution and impacts of climate change on the suitable habitats of Zingiber species in China. Ind Crop Prod 138:111429 ArticleGoogle Scholar
- Igawa T, Takahara T, Lau Q, Komaki S (2019) An application of PCR-RFLP species identification assay for environmental DNA detection. PeerJ 7:e7597 ArticleGoogle Scholar
- Inanaga M, Hasegawa Y, Mishima K, Takata K (2020) Genetic diversity and structure of Japanese endemic genus Thujopsis (Cupressaceae) using EST-SSR markers. Forests 11:935 ArticleGoogle Scholar
- IPBES (2019) The IPBES’ 2019 global assessment report on biodiversity and ecosystem services. UN Report: Nature’s Dangerous Decline “Unprecedented”; Species Extinction Rates “Accelerating.” In: United Nations Sustainable Development. https://www.un.org/sustainabledevelopment/blog/2019/05/nature-decline-unprecedentedreport. Accessed 7 Jul 2020
- Irina L-T, Javier B-P, Teresa C-BM, Eurídice L-A, María L, del Carmen C-I (2019) Integrating ecological and socioeconomic criteria in a GIS-based multicriteria-multiobjective analysis to develop sustainable harvesting strategies for Mexican oregano Lippia graveolens Kunth, a non-timber forest product. Land Use Policy 81:668–679 ArticleGoogle Scholar
- IUCN (2020) IUCN 2020. The IUCN red list of threatened species. Version 2020-1. In: IUCN red list of threatened species. https://www.iucnredlist.org/en. Accessed 6 Jul 2020
- Jahncke R, Leblon B, Bush P, LaRocque A (2018) Mapping wetlands in Nova Scotia with multi-beam RADARSAT-2 Polarimetric SAR, optical satellite imagery, and Lidar data. Int J Appl Earth Obs Geoinf 68:139–156 Google Scholar
- Jalal A, Salman A, Mian A, Shortis M, Shafait F (2020) Fish detection and species classification in underwater environments using deep learning with temporal information. Ecol Inform 57:101088 ArticleGoogle Scholar
- Jamil S, Fawad, Abbas MS et al (2020) Deep learning and computer vision-based a novel framework for himalayan bear, marco polo sheep and snow leopard detection. In: 2020 International Conference on Information Science and Communication Technology (ICISCT). IEEE, Karachi, Pakistan, pp 1–6
- Jansson S, Malmqvist E, Brydegaard M, Åkesson S, Rydell J (2020) A Scheimpflug lidar used to observe insect swarming at a wind turbine. Ecol Indic 117:106578 ArticleGoogle Scholar
- Jha SR, Naz R, Asif A, Okla MK, Soufan W, Al-Ghamdi AA, Ahmad A (2020) Development of an in vitro propagation protocol and a sequence characterized amplified region (SCAR) marker of Viola serpens Wall ex. Ging. Plants (Basel, Switzerland) 9:246 CASGoogle Scholar
- Karsli BA, Demir E, Fidan HG, Karsli T (2020) Assessment of genetic diversity and differentiation among four indigenous Turkish sheep breeds using microsatellites. Arch Anim Breed 63:165–172 ArticleGoogle Scholar
- Karthikeyan S, Preethi NSR (2018) (2018) Life detection system using UWB RADAR during disaster. Second Int Conf Green Comput Interne Things (ICGCIoT) 2:361–365 ArticleGoogle Scholar
- Kasprzak-Filipek K, Sawicka-Zugaj W, Litwińczuk Z, Chabuz W, Šveistienė R, Bulla J (2019) Assessment of the genetic structure of Central European cattle breeds based on functional gene polymorphism. Global Ecol Conserv 17:e00525 ArticleGoogle Scholar
- Khalighifar A, Brown RM, Goyes Vallejos J, Peterson AT (2021) Deep learning improves acoustic biodiversity monitoring and new candidate forest frog species identification (genus Platymantis) in the Philippines. Biodivers Conserv 30:643–657 ArticleGoogle Scholar
- Kim S-K (2019) Genetic diversity and DNA markers in fish. In: Kim S-K (ed) Essentials of Marine Biotechnology. Springer International Publishing, Cham, pp 109–144 ChapterGoogle Scholar
- Kim Y, Moon T (2016) Human detection and activity classification based on micro-doppler signatures using deep convolutional neural networks. IEEE Geosci Remote Sens Lett 13:8–12 ArticleCASGoogle Scholar
- Kittichai V, Pengsakul T, Chumchuen K, Samung Y, Sriwichai P, Phatthamolrat N, Tongloy T, Jaksukam K, Chuwongin S, Boonsang S (2021) Deep learning approaches for challenging species and gender identification of mosquito vectors. Sci Rep 11:4838 ArticleCASGoogle Scholar
- Klein DJ, McKown MW, Tershy BR (2015) Deep learning for large scale biodiversity monitoring. Bloomberg Data for Good, New York, p 7 Google Scholar
- Knapp N, Fischer R, Huth A (2018) Linking lidar and forest modeling to assess biomass estimation across scales and disturbance states. Remote Sens Environ 205:199–209 ArticleGoogle Scholar
- Knapp N, Fischer R, Cazcarra-Bes V, Huth A (2020) Structure metrics to generalize biomass estimation from lidar across forest types from different continents. Remote Sens Environ 237:111597 ArticleGoogle Scholar
- Kovács I, Tóth B, Schally G, Csányi S, Bleier N (2020) The assessment of wildlife damage estimation methods in maize with simulation in GIS environment. Crop Prot 127:104971 ArticleGoogle Scholar
- Koyama CN, Watanabe M, Hayashi M, Ogawa T, Shimada M (2019) Mapping the spatial-temporal variability of tropical forests by ALOS-2 L-band SAR big data analysis. Remote Sens Environ 233:111372 ArticleGoogle Scholar
- Krestoff ES, Creecy JP, Lord WD, Haynie ML, Coyer JA, Sampson K (2021) Mitochondrial DNA evaluation and species identification of Kemp’s Ridley Sea Turtle (Lepidochelys kempii) bones after a 3-year exposure to submerged marine and terrestrial environments. Front Mar Sci 8:646455 ArticleGoogle Scholar
- Krigas N, Papadimitriou K, Mazaris AD (2012) GIS and ex situ plant conservation. In: Alam BM (ed) Application of Geographic Information Systems. IntechOpen, London, SW1P 1WG, UK
- Kumar A, Kishore BSPC, Saikia P, Deka J, Bharali S, Singha LB, Tripathi OP, Khan ML (2019) Tree diversity assessment and above ground forests biomass estimation using SAR remote sensing: a case study of higher altitude vegetation of North-East Himalayas, India. Physics Chem Earth, Parts A/B/C 111:53–64 ArticleGoogle Scholar
- Kwok R (2019) AI empowers conservation biology. Nature 567:133–134 ArticleCASGoogle Scholar
- Kyrkjeeide MO, Westergaard KB, Kleven O, Evju M, Endrestøl A, Brandrud MK, Stabbetorp O (2020) Conserving on the edge: genetic variation and structure in northern populations of the endangered plant Dracocephalum ruyschiana L. (Lamiaceae). Conserv Genet 21:707–718 ArticleGoogle Scholar
- Labouisse J-P, Cubry P, Austerlitz F, Rivallan R, Nguyen HA (2020) New insights on spatial genetic structure and diversity of Coffea canephora(Rubiaceae) in Upper Guinea based on old herbaria. Plant Ecol Evol 153:82–100 ArticleGoogle Scholar
- Lambert M-J, Traoré PCS, Blaes X, Baret P, Defourny P (2018) Estimating smallholder crops production at village level from Sentinel-2 time series in Mali’s cotton belt. Remote Sens Environ 216:647–657 ArticleGoogle Scholar
- Lampert A (2019) Over-exploitation of natural resources is followed by inevitable declines in economic growth and discount rate. Nat Commun 10:1419 ArticleGoogle Scholar
- Lang N, Schindler K, Wegner JD (2019) Country-wide high-resolution vegetation height mapping with Sentinel-2. Remote Sens Environ 233:111347 ArticleGoogle Scholar
- Laurin GV, Puletti N, Grotti M, Stereńczak K, Modzelewska A, Lisiewicz M, Sadkowski R, Kuberski Ł, Chirici G, Papale D (2020) Species dominance and above ground biomass in the Białowieża Forest, Poland, described by airborne hyperspectral and lidar data. Int J Appl Earth Obs Geoinf 92:102178 Google Scholar
- Leapfrog (2021) GIS Data, Maps and Images. https://help.seequent.com/Geothermal/4.1/en-GB/Content/gisdata/gis-data.htm. Accessed 2 Aug 2021
- Lei Y, Treuhaft R, Keller M, dos-Santos M, Gonçalves F, Neumann M (2018) Quantification of selective logging in tropical forest with spaceborne SAR interferometry. Remote Sens Environ 211:167–183
- Li J, Zhao B, Chen Y, Zhao B, Yang N, Hu S, Shen J, Wu X (2020a) A genetic evaluation system for New Zealand white rabbit germplasm resources based on SSR markers. Animals : an Open Access Journal from MDPI 10:1258 ArticleGoogle Scholar
- Li W, Niu Z, Shang R, Qin Y, Wang L, Chen H (2020b) High-resolution mapping of forest canopy height using machine learning by coupling ICESat-2 LiDAR with Sentinel-1, Sentinel-2 and Landsat-8 data. Int J Appl Earth Obs Geoinf 92:102163 Google Scholar
- Liu L, Guo C, Li J, Xu H, Zhang J, Wang B (2016) Simultaneous life detection and localization using a wideband chaotic signal with an embedded tone. Sensors 16:1866 ArticleGoogle Scholar
- Liu J, Skidmore AK, Jones S, Wang T, Heurich M, Zhu X, Shi Y (2018) Large off-nadir scan angle of airborne LiDAR can severely affect the estimates of forest structure metrics. ISPRS J Photogramm Remote Sens 136:13–25 ArticleGoogle Scholar
- Liu F-M, Zhang N-N, Liu X-J, Yang Z-J, Jia H-Y, Xu D-P (2019a) Genetic diversity and population structure analysis of Dalbergia odorifera germplasm and development of a core collection using microsatellite markers. Genes 10
- Liu X, Faes L, Kale AU, Wagner SK, Fu DJ, Bruynseels A, Mahendiran T, Moraes G, Shamdas M, Kern C, Ledsam JR, Schmid MK, Balaskas K, Topol EJ, Bachmann LM, Keane PA, Denniston AK (2019b) A comparison of deep learning performance against health-care professionals in detecting diseases from medical imaging: A systematic review and meta-analysis. Lancet Digit Health 1:e271–e297 ArticleGoogle Scholar
- Liu J, Yong DL, Choi C-Y, Gibson L (2020) Transboundary frontiers: An emerging priority for biodiversity conservation. Trends Ecol Evol 35:679–690. https://doi.org/10.1016/j.tree.2020.03.004
- Lucas R, Van De Kerchove R, Otero V, Lagomasino D, Fatoyinbo L, Omar H, Satyanarayana B, Dahdouh-Guebas F (2020) Structural characterisation of mangrove forests achieved through combining multiple sources of remote sensing data. Remote Sens Environ 237:111543 ArticleGoogle Scholar
- Luo S, Wang C, Xi X, Pan F, Peng D, Zou J, Nie S, Qin H (2017) Fusion of airborne LiDAR data and hyperspectral imagery for aboveground and belowground forest biomass estimation. Ecol Indic 73:378–387 ArticleCASGoogle Scholar
- Ma J, Xiao X, Qin Y, Chen B, Hu Y, Li X, Zhao B (2017) Estimating aboveground biomass of broadleaf, needleleaf, and mixed forests in Northeastern China through analysis of 25-m ALOS/PALSAR mosaic data. For Ecol Manag 389:199–210 ArticleGoogle Scholar
- Ma Q, Su Y, Luo L, Li L, Kelly M, Guo Q (2018) Evaluating the uncertainty of Landsat-derived vegetation indices in quantifying forest fuel treatments using bi-temporal LiDAR data. Ecol Indic 95:298–310 ArticleGoogle Scholar
- Ma X, Mahecha MD, Migliavacca M, van der Plas F, Benavides R, Ratcliffe S, Kattge J, Richter R, Musavi T, Baeten L, Barnoaiea I, Bohn FJ, Bouriaud O, Bussotti F, Coppi A, Domisch T, Huth A, Jaroszewicz B, Joswig J et al (2019) Inferring plant functional diversity from space: the potential of Sentinel-2. Remote Sens Environ 233:111368 ArticleGoogle Scholar
- Malavasi M (2020) The map of biodiversity mapping. Biol Conserv 252:108843 ArticleGoogle Scholar
- Malik MH, Moaeen-Ud-Din M, Bilal G, Ghaffar A, Muner RD, Raja GK, Khan WA (2018) Development of amplified fragment length polymorphism (AFLP) markers for the identification of Cholistani cattle. Arch Anim Breed 61:387–394 ArticleGoogle Scholar
- Mammadov J, Aggarwal R, Buyyarapu R, Kumpatla S (2012) SNP Markers and their impact on plant breeding. Int J Plant Genomics 2012:728398 ArticleGoogle Scholar
- Manisalidis I, Stavropoulou E, Stavropoulos A, Bezirtzoglou E (2020) Environmental and health impacts of air pollution: a review. Front Public Health 8:14 ArticleGoogle Scholar
- Marchese C (2015) Biodiversity hotspots: a shortcut for a more complicated concept. Global Ecol Conserv 3:297–309 ArticleGoogle Scholar
- Martin-Abadal M, Ruiz-Frau A, Hinz H, Gonzalez-Cid Y (2020) Jellytoring: real-time jellyfish monitoring based on deep learning object detection. Sensors 20:1708 ArticleGoogle Scholar
- Martone M, Rizzoli P, Wecklich C, González C, Bueso-Bello J-L, Valdo P, Schulze D, Zink M, Krieger G, Moreira A (2018) The global forest/non-forest map from TandEM-X interferometric SAR data. Remote Sens Environ 205:352–373 ArticleGoogle Scholar
- Matasci G, Hermosilla T, Wulder MA, White JC, Coops NC, Hobart GW, Bolton DK, Tompalski P, Bater CW (2018) Three decades of forest structural dynamics over Canada’s forested ecosystems using Landsat time-series and lidar plots. Remote Sens Environ 216:697–714 ArticleGoogle Scholar
- McQuatters-Gollop A, Mitchell I, Vina-Herbon C, Bedford J, Addison PFE, Lynam CP, Geetha PN, Vermeulan EA, Smit K, Bayley DTI, Morris-Webb E, Niner HJ, Otto SA (2019) From science to evidence – how biodiversity indicators can be used for effective marine conservation policy and management. Front Mar Sci 6:109 ArticleGoogle Scholar
- Meena B, Singh N, Mahar KS, Sharma YK, Rana TS (2019) Molecular analysis of genetic diversity and population genetic structure in Ephedra foliata: an endemic and threatened plant species of arid and semi-arid regions of India. Physiol Mol Biol Plants: An International Journal of Functional Plant Biology 25:753–764 ArticleCASGoogle Scholar
- Mehring M, Mehlhaus N, Ott E, Hummel D (2020) A systematic review of biodiversity and demographic change: a misinterpreted relationship? Ambio 49:1297–1312 ArticleGoogle Scholar
- Mei Z, Khan MA, Zhang X, Fu J (2017) Rapid and accurate genetic authentication of Penthorum chinense by improved RAPD-derived species-specific SCAR markers. Biodivers J Biol Diver 18:1243–1249 ArticleGoogle Scholar
- Meikasari NS, Nurilmala M, Butet NA, Sudrajat AO (2019) PCR-RFLP as a detection method of allelic diversity seahorse Hippocampus comes (Cantor, 1849) from Bintan waters, Riau Island. IOP Conf Series: Earth Environ Sci 404:012046 Google Scholar
- Mercier A, Betbeder J, Baudry J, Le Roux V, Spicher F, Lacoux J, Roger D, Hubert-Moy L (2020) Evaluation of Sentinel-1 and 2 time series for predicting wheat and rapeseed phenological stages. ISPRS J Photogramm Remote Sens 163:231–256 ArticleGoogle Scholar
- Miller W, Hayes VM, Ratan A, Petersen DC, Wittekindt NE, Miller J, Walenz B, Knight J, Qi J, Zhao F, Wang Q, Bedoya-Reina OC, Katiyar N, Tomsho LP, Kasson LM, Hardie R-A, Woodbridge P, Tindall EA, Bertelsen MF et al (2011) Genetic diversity and population structure of the endangered marsupial Sarcophilus harrisii (Tasmanian devil). Proc Natl Acad Sci 108:12348–12353 ArticleCASGoogle Scholar
- Minh NTA, Van TT, Hau HV, Trieu LN, Tien CV, Vinh TT, Van DN (2019) Genetic diversity and variation of Huperzia serrata (Thunb. ex Murray) Trevis. population in Vietnam revealed by ISSR and SCoT markers. Biotechnol Biotechnol Equip 33:1525–1534 ArticleGoogle Scholar
- Mir AH, Tyub S, Kamili AN (2020) Ecology, distribution mapping and conservation implications of four critically endangered endemic plants of Kashmir Himalaya. Saudi J Biol Sci 27:2380–2389 ArticleGoogle Scholar
- Miraldo A, Li S, Borregaard MK, Flórez-Rodríguez A, Gopalakrishnan S, Rizvanovic M, Wang Z, Rahbek C, Marske KA, Nogués-Bravo D (2016) An anthropocene map of genetic diversity. Science 353:1532–1535 ArticleCASGoogle Scholar
- Molerović N, Rašković B, Đedović R, Andrić OD, Marković Z, Marić S (2019) Characterization of the genetic structure of the brown trout (Salmo trutta) from “Braduljica” fish farm, Serbia. Biotechnol Anim Husband 35:289–299 ArticleGoogle Scholar
- Mosa KA, Gairola S, Jamdade R, El-Keblawy A, Al Shaer KI, Al Harthi EK, Shabana HA, Mahmoud T (2019) The promise of molecular and genomic techniques for biodiversity research and DNA barcoding of the arabian peninsula flora. Front Plant Sci 9:1929 ArticleGoogle Scholar
- Mudgineer O jpg: *derivative work: (2011) Oil drilling rig, simple illustration. https://commons.wikimedia.org/wiki/File:Oil_Rig_NT8.svg. Accessed 9 Aug 2021
- Naczk AM and Ziętara MS (2019) Genetic diversity in Dactylorhiza majalis subsp. majalis populations (Orchidaceae) of northern Poland. Nordic J Bot 37. https://doi.org/10.1111/njb.01989
- Naeem S, Prager C, Weeks B, Varga A, Flynn DFB, Griffin K, Muscarella R, Palmer M, Wood S, Schuster W (2016) Biodiversity as a multidimensional construct: a review, framework and case study of herbivory’s impact on plant biodiversity. Proc Biol Sci 283:20153005 Google Scholar
- Najafzadeh R, Arzani K, Bouzari N, Saei A (2014) Genetic diversity assessment and identification of new sour cherry genotypes using intersimple sequence repeat markers. Int J Biodivers 2o14:308398 Google Scholar
- Neiber MT, Cianfanelli S, Bartolini F, Glaubrecht M (2020) Not a marginal loss: genetic diversity of the endangered freshwater snail Melanopsis etrusca (Brot, 1862) from thermal springs in Tuscany, Italy. Conserv Genet 21:199–216 ArticleGoogle Scholar
- Neugarten RA, Langhammer PF, Osipova E et al (2018) Tools for measuring, modelling, and valuing ecosystem services: guidance for key biodiversity areas, natural world heritage sites, and protected areas, 1st edn. (ed. by Groves C) IUCN, International Union for Conservation of Nature, USA
- Neugarten RA, Moull K, Martinez NA, Andriamaro L, Bernard C, Bonham C, Cano CA, Ceotto P, Cutter P, Farrell TA, Gibb M, Goedschalk J, Hole D, Honzák M, Kasecker T, Koenig K, Larsen TH, Ledezma JC, McKinnon M et al (2020) Trends in protected area representation of biodiversity and ecosystem services in five tropical countries. Ecosyst Serv 42:101078 ArticleGoogle Scholar
- Newbold T, Hudson LN, Hill SLL, Contu S, Lysenko I, Senior RA, Börger L, Bennett DJ, Choimes A, Collen B, Day J, De Palma A, Díaz S, Echeverria-Londoño S, Edgar MJ, Feldman A, Garon M, Harrison MLK, Alhusseini T et al (2015) Global effects of land use on local terrestrial biodiversity. Nature 520:45–50 ArticleCASGoogle Scholar
- Ng WL, Tan SG (2015) Inter-Simple Sequence Repeat (ISSR) markers: are we doing it right? ASM Sci J 9:30–39 Google Scholar
- Omasa K, Hosoi F, Konishi A (2007) 3D lidar imaging for detecting and understanding plant responses and canopy structure. J Exp Bot 58:881–898 ArticleCASGoogle Scholar
- Oon A, Ngo KD, Azhar R, Ashton-Butt A, Lechner AM, Azhar B (2019) Assessment of ALOS-2 PALSAR-2L-band and Sentinel-1 C-band SAR backscatter for discriminating between large-scale oil palm plantations and smallholdings on tropical peatlands. Remote Sens Appl: Soc Environ 13:183–190 Google Scholar
- Organikos (2012) Thermal imaging, elephant listening. In: Organikos. https://organikos.net/2012/09/23/thermalimaging-elephant-listening/. Accessed 9 Aug 2021
- Our World In Data (2020) Forest area as share of land area, Source: UN Food and Agriculture Organization (FAO). https://commons.wikimedia.org/wiki/File:Forest_area_as_share_of_land_area,_OWID.svg. Accessed 9 Aug 2021
- Özdil F, İlhan F, Işık R (2018) Genetic characterization of some Turkish sheep breeds based on the sequencing of the Ovar-DRB1 gene in the major histocompatibility complex (MHC) gene region. Arch Anim Breed 61:475–480 ArticleGoogle Scholar
- Özdil F, Bulut H, Işık R (2019) Genetic diversity of κ-casein (CSN3) and lactoferrin (LTF) genes in the endangered Turkish donkey (Equus asinus) populations. Arch Anim Breed 62:77–82 ArticleGoogle Scholar
- Panicz R, Napora-Rutkowski Ł, Keszka S, Skuza L, Szenejko M, Śmietana P (2019) Genetic diversity in natural populations of noble crayfish (Astacus astacus L.) in north-western Poland on the basis of combined SSR and AFLP data. PeerJ 7:e7301 ArticleGoogle Scholar
- Panigrahi S, Velraj P, Subba Rao T (2019) Chapter 21 - Functional microbial diversity in contaminated environment and application in bioremediation. In: Das S, Dash HR (eds) Microbial Diversity in the Genomic Era. Academic Press, London, pp 359–385 ChapterGoogle Scholar
- Parrens M, Bitar AA, Frappart F, Paiva R, Wongchuig S, Papa F, Yamasaki D, Kerr Y (2019) High resolution mapping of inundation area in the Amazon basin from a combination of L-band passive microwave, optical and radar datasets. Int J Appl Earth Obs Geoinf 81:58–71 Google Scholar
- Parthiban S, Govindaraj P, Senthilkumar S (2018) Comparison of relative efficiency of genomic SSR and EST-SSR markers in estimating genetic diversity in sugarcane. 3. Biotech 8:144 CASGoogle Scholar
- Pixabay (2021) 110,000+ free vector stock art images, hand selected - pixabay. https://pixabay.com/vectors/. Accessed 11 Aug 2021
- Pngtree (2021) Millions of PNG images, backgrounds and vectors for free download. In: Pngtree. href='https://pngtree.com/so/shouting-horn'> shouting horn png from pngtree.com. Accessed 2 Aug 2021
- Portree D (2006) A diagram showing the orbital configuration of an Almaz radar satellite, a type of Soviet reconnaissance satellite based on the Almaz OPS space stations. https://commons.wikimedia.org/wiki/File:Almaz_radar_satellite.svg. Accessed 9 Aug 2021
- Prošek J, Gdulová K, Barták V, Vojar J, Solský M, Rocchini D, Moudrý V (2020) Integration of hyperspectral and LiDAR data for mapping small water bodies. Int J Appl Earth Obs Geoinf 92:102181 Google Scholar
- Qamer S, Al-Abbadi AA, Sajid M, Asad F, Khan MF, Khan NA, Sthanadar AA, Akhtar MN, Mahmoud AH, Mohammed OB (2021) Genetic analysis of honey bee, Apis dorsata populations using random amplified polymorphic DNA (RAPD) markers. J King Saud Univ - Sci 33:101218 ArticleGoogle Scholar
- Qi W, Saarela S, Armston J, Ståhl G, Dubayah R (2019) Forest biomass estimation over three distinct forest types using TandEM-X InSAR data and simulated GEDI lidar data. Remote Sens Environ 232:111283 ArticleGoogle Scholar
- Qiao Y, Guo F, Huo N, Zhan L, Sun J, Zuo X, Guo Z, Gu YQ, Wang Y, Liu Y (2021) Genotyping-by-sequencing to determine the genetic structure of a Tibetan medicinal plant Swertia mussotii Franch. Genet Resour Crop Evol 68:469–484 ArticleCASGoogle Scholar
- Rajah P, Odindi J, Mutanga O (2018) Feature level image fusion of optical imagery and Synthetic Aperture Radar (SAR) for invasive alien plant species detection and mapping. Remote Sens Appl: Soc Environ 10:198–208 Google Scholar
- Randin CF, Ashcroft MB, Bolliger J, Cavender-Bares J, Coops NC, Dullinger S, Dirnböck T, Eckert S, Ellis E, Fernández N, Giuliani G, Guisan A, Jetz W, Joost S, Karger D, Lembrechts J, Lenoir J, Luoto M, Morin X et al (2020) Monitoring biodiversity in the Anthropocene using remote sensing in species distribution models. Remote Sens Environ 239:111626 ArticleGoogle Scholar
- Rappaport DI, Royle JA, Morton DC (2020) Acoustic space occupancy: combining ecoacoustics and lidar to model biodiversity variation and detection bias across heterogeneous landscapes. Ecol Indic 113:106172 ArticleGoogle Scholar
- Ray A, Jena S, Haldar T, Sahoo A, Kar B, Patnaik J, Ghosh B, Chandra Panda P, Mahapatra N, Nayak S (2019) Population genetic structure and diversity analysis in Hedychium coronarium populations using morphological, phytochemical and molecular markers. Ind Crop Prod 132:118–133 ArticleCASGoogle Scholar
- Reeth CV, Michel N, Bockstaller C, Caro G (2019) Influences of oilseed rape area and aggregation on pollinator abundance and reproductive success of a co-flowering wild plant. Agric Ecosyst Environ 280:35–42 ArticleGoogle Scholar
- Reid AJ, Carlson AK, Creed IF, Eliason EJ, Gell PA, Johnson PTJ, Kidd KA, MacCormack TJ, Olden JD, Ormerod SJ, Smol JP, Taylor WW, Tockner K, Vermaire JC, Dudgeon D, Cooke SJ (2019) Emerging threats and persistent conservation challenges for freshwater biodiversity. Cambridge Philos Soc 94:849–873 ArticleGoogle Scholar
- Ridley FA, McGowan PJ, Mair L (2020) The scope and extent of literature that maps threats to species: a systematic map protocol. Environ Evid 9:23 ArticleGoogle Scholar
- Righi T, Splendiani A, Fioravanti T, Petetta A, Candelma M, Gioacchini G, Gillespie K, Hanke A, Carnevali O, Caputo Barucchi V (2020) Mediterranean swordfish (Xiphias gladius Linnaeus, 1758) population structure revealed by microsatellite DNA: genetic diversity masked by population mixing in shared areas. PeerJ 8:e9518 ArticleGoogle Scholar
- Rodríguez-Peña RA, Johnson RL, Johnson LA, Anderson CD, Ricks NJ, Farley KM, Robbins MD, Wolfe AD, Stevens MR (2018) Investigating the genetic diversity and differentiation patterns in the Penstemon scariosus species complex under different sample sizes using AFLPs and SSRs. Conserv Genet 19:1335–1348 ArticleGoogle Scholar
- Rudd S (2003) Expressed sequence tags: alternative or complement to whole genome sequences? Trends Plant Sci 8:321–329 ArticleCASGoogle Scholar
- Saikia M, Devi D (2019) Analysis of genetic diversity and phylogeny of Philosamia ricini (Lepidoptera: Saturniidae) by using RAPD and internal transcribed spacer DNA1. Mol Biol Rep 46:3035–3048 ArticleCASGoogle Scholar
- Saikia M, Haloi K, Nath R, Devi D (2019) Genetic diversity among the morphs of Antheraea assamensis Helfer: study using RAPD and internal transcribed spacer DNA1. Indian J Exp Biol 57:418–426 CASGoogle Scholar
- Sairkar PK, Sharma A, Shukla NP (2016) SCAR marker for identification and discrimination of Commiphora wightii and C. myrrha. Mol Biol Int 2016:1482796. https://doi.org/10.1155/2016/1482796
- Saleem MH, Potgieter J, Arif KM (2019) Plant disease detection and classification by deep learning. Plants 8:468 ArticleGoogle Scholar
- Saleem MH, Potgieter J, Arif KM (2020) Plant disease classification: a comparative evaluation of convolutional neural networks and deep learning optimizers. Plants 9:1319 ArticleGoogle Scholar
- Salehi F, Ahmadian L (2017) The application of geographic information systems (GIS) in identifying the priority areas for maternal care and services. BMC Health Serv Res 17:482 ArticleGoogle Scholar
- Sarwat M (2012) ISSR: a reliable and cost-effective technique for detection of DNA polymorphism. In: Sucher NJ, Hennell JR, Carles MC (eds) Plant DNA Fingerprinting and Barcoding: Methods and Protocols Methods in Molecular Biology. Humana Press, Totowa, pp 103–121 ChapterGoogle Scholar
- Schägner JP, Brander L, Maes J, Hartje V (2013) Mapping ecosystem services’ values: current practice and future prospects. Ecosyst Serv 4:33–46 ArticleGoogle Scholar
- Schlund M, Erasmi S (2020) Sentinel-1 time series data for monitoring the phenology of winter wheat. Remote Sens Environ 246:111814 ArticleGoogle Scholar
- Scionti F, Di Martino MT, Pensabene L, Bruni V, Concolino D (2018) The cytoscan HD array in the diagnosis of neurodevelopmental disorders. High-Throughput 7:E28 ArticleGoogle Scholar
- Sedano F, Lisboa S, Duncanson L, Ribeiro N, Sitoe A, Sahajpal R, Hurtt G, Tucker C (2020) Monitoring intra and inter annual dynamics of forest degradation from charcoal production in Southern Africa with Sentinel – 2 imagery. Int J Appl Earth Obs Geoinf 92:102184 Google Scholar
- Selvaraj MG, Vergara A, Montenegro F, Alonso Ruiz H, Safari N, Raymaekers D, Ocimati W, Ntamwira J, Tits L, Omondi AB, Blomme G (2020) Detection of banana plants and their major diseases through aerial images and machine learning methods: a case study in DR Congo and Republic of Benin. ISPRS J Photogramm Remote Sens 169:110–124 ArticleGoogle Scholar
- Senn HV, Ghazali M, Kaden J, Barclay D, Harrower B, Campbell RD, Macdonald DW, Kitchener AC (2019) Distinguishing the victim from the threat: SNP-based methods reveal the extent of introgressive hybridization between wildcats and domestic cats in Scotland and inform future in situ and ex situ management options for species restoration. Evol Appl 12:399–414 ArticleGoogle Scholar
- Sereshkeh FM, Azizi A, Noroozisharaf A (2019) Structure of genetic diversity among and within populations of the endemic Iranian plant Dracocephalum kotschyi. Hortic Environ Biotechnol 60:767–777 ArticleGoogle Scholar
- Shen L, Li X-W, Meng X-X, Wu J, Tang H, Huang L-F, Xiao S-M, Xu J, Chen S-L (2019) Prediction of the globally ecological suitability of Panax quinquefolius by the geographic information system for global medicinal plants (GMPGIS). Chin J Nat Med 17:481–489 CASGoogle Scholar
- Silvestro D, Goria S, Sterner T, Antonelli A (2022) Improving biodiversity protection through artificial intelligence. Nat Sustain 5:415–424 ArticleGoogle Scholar
- Slagter B, Tsendbazar N-E, Vollrath A, Reiche J (2020) Mapping wetland characteristics using temporally dense Sentinel-1 and Sentinel-2 data: a case study in the St. Lucia wetlands, South Africa. Int J Appl Earth Obs Geoinf 86:102009 Google Scholar
- Smithsonian’s National Zoo and Conservation Biology Institute Smithsonian’s National Zoo (2016) Asian elephant. In: Smithsonian’s National Zoo. https://nationalzoo.si.edu/animals/asian-elephant. Accessed 9 Aug 2021
- Srikanth K, Kim N-Y, Park W, Kim J-M, Kim K-D, Lee K-T, Son J-H, Chai H-H, Choi J-W, Jang G-W, Kim H, Ryu Y-C, Nam J-W, Park J-E, Kim J-M, Lim D (2019) Comprehensive genome and transcriptome analyses reveal genetic relationship, selection signature, and transcriptome landscape of small-sized Korean native Jeju horse. Sci Rep 9:16672 ArticleGoogle Scholar
- Srivastava PK, Malhi RKM, Pandey PC, Anand A, Singh P, Pandey MK, Gupta A (2020) 1 - Revisiting hyperspectral remote sensing: origin, processing, applications and way forward. In: Pandey PC, Srivastava PK, Balzter H, Bhattacharya B, Petropoulos GP (eds) Hyperspectral Remote Sensing Earth Observation. Elsevier, Amsterdam, pp 3–21 ChapterGoogle Scholar
- Stephenson PJ (2020) Technological advances in biodiversity monitoring: applicability, opportunities and challenges. Curr Opin Environ Sustain 45:36–41 ArticleGoogle Scholar
- Sulistyahadi FN, Puspitasari IGAAR, Nuryanto A (2020) Diversity analysis of Rhacophorus margaritifer (Schlegel, 1837) in Baturraden based on RAPD markers. J Trop Biodiversi Biotechnol 5:44–52 ArticleGoogle Scholar
- Sun Y-W, Hou N, Woeste K, Zhang C, Yue M, Yuan X-Y, Zhao P (2019) Population genetic structure and adaptive differentiation of iron walnut Juglans regia subsp. sigillata in southwestern China. Ecol Evol 9:14154–14166 ArticleGoogle Scholar
- Supple MA, Shapiro B (2018) Conservation of biodiversity in the genomics era. Genome Biol 19:131 ArticleGoogle Scholar
- Tanase MA, Villard L, Pitar D, Apostol B, Petrila M, Chivulescu S, Leca S, Borlaf-Mena I, Pascu I-S, Dobre A-C, Pitar D, Guiman G, Lorent A, Anghelus C, Ciceu A, Nedea G, Stanculeanu R, Popescu F, Aponte C, Badea O (2019) Synthetic aperture radar sensitivity to forest changes: a simulations-based study for the Romanian forests. Sci Total Environ 689:1104–1114 ArticleCASGoogle Scholar
- Tani N, Kawahara T, Yoshimaru H, Hoshi Y (2003) Development of SCAR markers distinguishing pure seedlings of the endangered species Morus boninensis from M. boninensis × M. acidosa hybrids for conservation in Bonin (Ogasawara) Islands. Conserv Genet 4:605–612 ArticleCASGoogle Scholar
- Tarazona Y, Miyasiro-López M (2020) Monitoring tropical forest degradation using remote sensing. Challenges and opportunities in the Madre de Dios region, Peru. Remote Sens Appl: Soc Environ 19:100337 Google Scholar
- Tashayo B, Honarbakhsh A, Akbari M, Eftekhari M (2020) Land suitability assessment for maize farming using a GIS-AHP method for a semi- arid region, Iran. J Saudi Soc Agric Sci 19(5):332–338 Google Scholar
- Teobaldelli M, Cona F, Saulino L, Migliozzi A, D’Urso G, Langella G, Manna P, Saracino A (2017) Detection of diversity and stand parameters in Mediterranean forests using leaf-off discrete return LiDAR data. Remote Sens Environ 192:126–138 ArticleGoogle Scholar
- The RAFOS group at the Graduate School of Oceanography, University of Rhode Island, Kingston, RI 02881 (2001) The ideal signal received from moored SOFAR emitters and several recorded signals from the float. The arrival time can be measured very accurately. https://commons.wikimedia.org/wiki/File:Sound_wave_Correlation.jpg. Accessed 11 Aug 2021
- Thessen A (2016) Adoption of machine learning techniques in ecology and earth science. One Ecosyst 1:e8621 ArticleGoogle Scholar
- Thuy MTP, Ha TTT, Quang TH (2020) Analysis of genetic diversity in Pa Co pine (Pinus kwangtungensis Chun ex Tsiang) using RAPD and ISSR markers. Vietnam J Sci Technol Eng 62:62–68 ArticleGoogle Scholar
- Tilman D, Isbell F, Cowles JM (2014) Biodiversity and ecosystem functioning. Annu Rev Ecol Evol Syst 45:471–493 ArticleGoogle Scholar
- Tiwari V, Meena B, Nair NK, Rana TS (2020) Molecular analyses of genetic variability in the populations of Bergenia ciliata in Indian Himalayan Region (IHR). Physiol Mol Biol Plants: An International Journal of Functional Plant Biology 26:975–984 ArticleGoogle Scholar
- Torresani M, Rocchini D, Sonnenschein R, Zebisch M, Hauffe HC, Heym M, Pretzsch H, Tonon G (2020) Height variation hypothesis: a new approach for estimating forest species diversity with CHM LiDAR data. Ecol Indic 117:106520 ArticleGoogle Scholar
- Touma S, Arakawa A, Oikawa T (2019) Evaluation of the genetic structure of indigenous Okinawa Agu pigs using microsatellite markers. Asian Australas J Anim Sci 33:212–218 ArticleGoogle Scholar
- Tuisima-Coral LL, Hlásná Čepková P, Weber JC, Lojka B (2020) Preliminary evidence for domestication effects on the genetic diversity of Guazuma crinita in the Peruvian Amazon. Forests 11:795 ArticleGoogle Scholar
- Valbuena R, O’Connor B, Zellweger F, Simonson W, Vihervaara P, Maltamo M, Silva CA, Almeida DRA, Danks F, Morsdorf F, Chirici G, Lucas R, Coomes DA, Coops NC (2020) Standardizing ecosystem morphological traits from 3D information sources. Trends Ecol Evol 35(8):656–667 ArticleCASGoogle Scholar
- Vallejos-Vidal E, Reyes-Cerpa S, Rivas-Pardo JA, Maisey K, Yáñez JM, Valenzuela H, Cea PA, Castro-Fernandez V, Tort L, Sandino AM, Imarai M, Reyes-López FE (2019) Single-nucleotide polymorphisms (SNP) mining and their effect on the tridimensional protein structure prediction in a set of immunity-related expressed sequence tags (EST) in Atlantic salmon (Salmo salar). Front Genet 10:1406 ArticleCASGoogle Scholar
- Van den Broeck T, Joniau S, Clinckemalie L, Helsen C, Prekovic S, Spans L, Tosco L, Van Poppel H, Claessens F (2014) The role of single nucleotide polymorphisms in predicting prostate cancer risk and therapeutic decision making. Biomed Res Int 2014:627510 Google Scholar
- Vaux F, Aycock HM, Bohn S, Rasmuson LK, O’Malley KG (2020) Sex identification PCR–RFLP assay tested in eight species of Sebastes rockfish. Conserv Genet Resour 12:541–544 ArticleGoogle Scholar
- Vecteezy (2021a) Set of wild animal. In: Vecteezy.com. href https://www.vecteezy.com/freevector/vector" data-track="click||click_references" data-track-action="external reference" data-track-value="external reference" data-track-label="https://www.vecteezy.com/freevector/vector">https://www.vecteezy.com/freevector/vector"> Vector Vectors by Vecteezy. Accessed 25 May 2021
- Vecteezy (2021b) Forest scene with tall trees. In: Vecteezy.com. https://www.vecteezy.com/vector-art/298788-forestscene-with-tall-trees. Accessed 25 May 2021
- Vergnaud G, Denoeud F (2000) Minisatellites: mutability and genome architecture. Genome Res 10:899–907 ArticleCASGoogle Scholar
- Verma GK, Gupta P (2018) Wild animal detection using deep convolutional neural network. In: Chaudhuri BB, Kankanhalli MS, Raman B (eds) Proceedings of 2nd International Conference on Computer Vision and Image Processing Advances in Intelligent Systems and Computing. Springer, Singapore, pp 327–338 Google Scholar
- Vidaña-Vila E, Navarro J, Alsina-Pagès RM, Ramírez Á (2020) A two-stage approach to automatically detect and classify woodpecker (Fam. Picidae) sounds. Appl Acoust 166:107312 ArticleGoogle Scholar
- Vieira MLC, Santini L, Diniz AL, de Freitas Munhoz C (2016) Microsatellite markers: what they mean and why they are so useful. Genet Mol Biol 39:312–328 ArticleGoogle Scholar
- Vignal A, Milan D, SanCristobal M, Eggen A (2002) A review on SNP and other types of molecular markers and their use in animal genetics. Gen Select Evol: GSE 34:275–305 ArticleCASGoogle Scholar
- Vogeler JC, Cohen WB (2016) A review of the role of active remote sensing and data fusion for characterizing forest in wildlife habitat models. Span J Remote Sens 45:1–14 Google Scholar
- Wagutu GK, Fan X-R, Njeri HK, Wen X-Y, Liu Y-L, Chen Y-Y (2020) Development and characterization of EST-SSR markers for the endangered tree Magnolia patungensis (Magnoliaceae). Ann Bot Fenn 57:97–107 ArticleGoogle Scholar
- Wang R, Gamon JA (2019) Remote sensing of terrestrial plant biodiversity. Remote Sens Environ 231:111218 ArticleGoogle Scholar
- Wang J, Xiao X, Qin Y, Doughty RB, Dong J, Zou Z (2018) Characterizing the encroachment of juniper forests into sub-humid and semi-arid prairies from 1984 to 2010 using PALSAR and Landsat data. Remote Sens Environ 205:166–179 ArticleGoogle Scholar
- Wang J, Xiao X, Bajgain R, Starks P, Steiner J, Doughty RB, Chang Q (2019a) Estimating leaf area index and aboveground biomass of grazing pastures using Sentinel-1, Sentinel-2 and Landsat images. ISPRS J Photogramm Remote Sens 154:189–201 ArticleGoogle Scholar
- Wang X, Chen W, Luo J, Yao Z, Yu Q, Wang Y, Zhang S, Liu Z, Zhang M, Shen Y (2019b) Development of EST-SSR markers and their application in an analysis of the genetic diversity of the endangered species Magnolia sinostellata. Mol Gen Genomics: MGG 294:135–147 ArticleCASGoogle Scholar
- Wang L, Deng H, Qiu X, Wang P, Yang F (2020) Determining the impact of key climatic factors on geographic distribution of wild Akebia trifoliata. Ecol Indic 112:106093 ArticleGoogle Scholar
- Wetzel FT, Saarenmaa H, Regan E, Martin CS, Mergen P, Smirnova L, Tuama ÉÓ, Camacho FAG, Hoffmann A, Vohland K, Häuser CL (2015) The roles and contributions of Biodiversity Observation Networks (BONs) in better tracking progress to 2020 biodiversity targets: a European case study. Biodiversity 16:137–149 ArticleGoogle Scholar
- Whitehorn PR, Navarro LM, Schröter M, Fernandez M, Rotllan-Puig X, Marques A (2019) Mainstreaming biodiversity: a review of national strategies. Biol Conserv 235:157–163 ArticleGoogle Scholar
- Whyte A, Ferentinos KP, Petropoulos GP (2018) A new synergistic approach for monitoring wetlands using Sentinels-1 and 2 data with object-based machine learning algorithms. Environ Model Softw 104:40–54 ArticleGoogle Scholar
- Willi M, Pitman RT, Cardoso AW, Locke C, Swanson A, Boyer A, Veldthuis M, Fortson L (2018) Software, data and models used in “Identifying animal species in camera trap images using deep learning and citizen science
- Willi M, Pitman RT, Cardoso AW, Locke C, Swanson A, Boyer A, Veldthuis M, Fortson L (2019) Identifying animal species in camera trap images using deep learning and citizen science. Methods Ecol Evol 10:80–91 ArticleGoogle Scholar
- Wu K, Rodriguez GA, Zajc M, Jacquemin E, Clément M, De Coster A, Lambot S (2019a) A new drone-borne GPR for soil moisture mapping. Remote Sens Environ 235:111456 ArticleGoogle Scholar
- Wu W-D, Liu W-H, Sun M, Zhou J-Q, Liu W, Zhang C-L, Zhang X-Q, Peng Y, Huang L-K, Ma X (2019b) Genetic diversity and structure of Elymus tangutorum accessions from western China as unraveled by AFLP markers. Hereditas 156:8 ArticleGoogle Scholar
- Xia D, Chen P, Wang B, Zhang J, Xie C (2018) Insect detection and classification based on an improved convolutional neural network. Sensors, Basel, p 18 Google Scholar
- Xia W, Luo T, Zhang W, Mason AS, Huang D, Huang X, Tang W, Dou Y, Zhang C, Xiao Y (2019) Development of high density SNP markers and their application in evaluating genetic diversity and population structure in Elaeis guineensis. Front Plant Sci 10:130 ArticleGoogle Scholar
- Xu F, Lei P, Jiang M, Sang L, Guan F, Meng F, Quan H (2019) Genetic diversity of Herpetospermum caudigerum (Ser.) Baill using AFLP and chloroplast microsatellites. Biotechnol Biotechnol Equip 33:1260–1268 ArticleGoogle Scholar
- Yang L, Khan MA, Mei Z, Yang M, Zhang T, Wei C, Yang W, Zhu L, Long Y, Fu J (2014) Development of RAPD-SCAR markers for Lonicera japonica (Caprifolicaceae) variety authentication by improved RAPD and DNA cloning. Revista De Biol Trop 62:1649–1657 ArticleGoogle Scholar
- Yin L and Zhou YM (2019) Life detection strategy based on infrared vision and ultra-wideband radar data fusion. Elect Eng Syst Sci 1–7
- Yuskianti V, Shiraishi S (2010) Sequence characterized amplified region (SCAR) markers in Sengon (Paraseriathes falcataria (L.)) Nielsen. Hayati J Biosci 17:167–172 ArticleGoogle Scholar
- Zeng Z, Gan Y, Kettner AJ, Yang Q, Zeng C, Brakenridge GR, Hong Y (2020) Towards high resolution flood monitoring: an integrated methodology using passive microwave brightness temperatures and Sentinel synthetic aperture radar imagery. J Hydrol 582:124377 ArticleGoogle Scholar
- Zhang C, Patras P, Haddadi H (2019a) Deep learning in mobile and wireless networking: a survey. IEEE Commun Surv Tutor 21:2224–2287 ArticleGoogle Scholar
- Zhang P, Nascetti A, Ban Y, Gong M (2019b) An implicit radar convolutional burn index for burnt area mapping with Sentinel-1 C-band SAR data. ISPRS J Photogramm Remote Sens 158:50–62 ArticleGoogle Scholar
- Zhang W, Brandt M, Wang Q, Prishchepov AV, Tucker CJ, Li Y, Lyu H, Fensholt R (2019c) From woody cover to woody canopies: how Sentinel-1 and Sentinel-2 data advance the mapping of woody plants in savannas. Remote Sens Environ 234:111465 ArticleGoogle Scholar
- Zhang Y, Ling F, Foody GM, Ge Y, Boyd DS, Li X, Du Y, Atkinson PM (2019d) Mapping annual forest cover by fusing PALSAR/PALSAR-2 and MODIS NDVI during 2007–2016. Remote Sens Environ 224:74–91 ArticleGoogle Scholar
- Zhang Y, Zhang M, Hu Y, Zhuang X, Xu W, Li P, Wang Z (2019e) Mining and characterization of novel EST-SSR markers of Parrotia subaequalis (Hamamelidaceae) from the first Illumina-based transcriptome datasets. PLoS One 14:e0215874 ArticleGoogle Scholar
- Zheng Y, Lan S, Chen WY, Chen X, Xu X, Chen Y, Dong J (2019) Visual sensitivity versus ecological sensitivity: an application of GIS in urban forest park planning. Urban For Urban Green 41:139–149 ArticleGoogle Scholar
- Zhu X, Hou Y, Weng Q, Chen L (2019) Integrating UAV optical imagery and LiDAR data for assessing the spatial relationship between mangrove and inundation across a subtropical estuarine wetland. ISPRS J Photogramm Remote Sens 149:146–156 ArticleGoogle Scholar
- Zimmermann BL, De Vargas Machado JV, Santos S, Bartholomei-Santos ML (2019) Genetic diversity of three aegla species (Decapoda, Anomura) revealed by AFLP and mtDNA markers. Crustaceana 19:445–462 ArticleGoogle Scholar
- Zizka A, Silvestro D, Vitt P, Knight TM (2020) Automated conservation assessment of the orchid family with deep learning. Conserv Biol 35:897–908 n/a ArticleGoogle Scholar
Author information
Authors and Affiliations
- Department of Biotechnology, Utkal University, Vani Vihar, Bhubaneswar, Odisha, 751004, India Rout George Kerry & Sanatan Majhi
- College of Informatics and Computing Sciences, Batangas State University, Batangas, Philippines Francis Jesmar Perez Montalbo
- Department of Soil Science and Agricultural Chemistry, School of Agriculture, GIET University, Gunupur, Rayagada, Odisha, 765022, India Rajeswari Das
- Indian Council of Agricultural Research-Directorate of Foot and Mouth Disease-International Centre for Foot and Mouth Disease, Arugul, Bhubaneswar, Odisha, 752050, India Sushmita Patra & Vinayak Nayak
- Department of Anatomy and Cell Biology, McGill University, Montreal, Quebec, H3A 0C7, Canada Gyana Prakash Mahapatra
- Zoology Section, Mahila MahaVidyalya, Banaras Hindu University, Varanasi, 221005, India Ganesh Kumar Maurya
- Department of Neurosurgery, Brigham and Women’s Hospital, Harvard Medical School, Boston, MA, 02115, USA Atala Bihari Jena
- Department of Physics, Edo State University Uzairue, P.B.M. 04, Auchi, Edo State, 312101, Nigeria Kingsley Eghonghon Ukhurebor
- Department of Pharmaceutical Sciences, Utkal University, Vani Vihar, Bhubaneswar, Odisha, 751004, India Ram Chandra Jena
- Department of Zoology, Mizoram University, Aizawl, 796009, India Sushanto Gouda
- School of Biological Sciences, AIPH University, Bhubaneswar, Odisha, 752101, India Jyoti Ranjan Rout
- Rout George Kerry